Gene Regulation PDF
Document Details
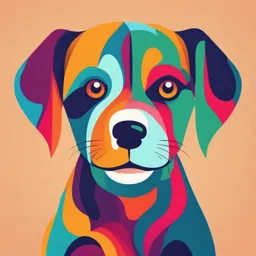
Uploaded by .keeks.
Marian University
Tags
Related
- Molecular Biology of Gene Regulation PDF
- Biology Chapter 18 Lecture Notes (gene expression) PDF
- Processes Test 3 PDF
- Cell and Molecular Biology Lecture 35: Gene Regulation in Eukaryotes PDF
- The Human Body PJ1311 Central Dogma of Life (Transcription and Translation) PDF
- 5th Course Gene Regulation 1 PDF
Summary
This document discusses gene regulation, particularly at the level of transcription in eukaryotes. It explains how genes are controlled and regulated and details the different levels of regulation. It also describes various pathways and processes involved like transcription factors, RNA polymerase and micro-RNAs.
Full Transcript
We're gonna take a quick little side step. Eukaryotes predominantly regulate at the level of transcription. This is different than our friends. If a gene is super important to daily life, to fundamental biochemical activity, to breathing, if you will, what's it likely to be? Always on, right? Consti...
We're gonna take a quick little side step. Eukaryotes predominantly regulate at the level of transcription. This is different than our friends. If a gene is super important to daily life, to fundamental biochemical activity, to breathing, if you will, what's it likely to be? Always on, right? Constitutively expressed. You need it in everything you do, chances are that gene is on. We as an organism try to control things by turning them on rather than turning them off but we still have some things that in theory should always be on. So because of that, we have different levels of expression that run along that same molecular genetics that we had been talking about. While transcription is our predominant mechanism, it is not the sole mechanism. Post-transcriptional regulation is critical. Failures in processing, failures in compartmentalization, moving things to the right compartment, so localization, failures in export, failures in import from the external environment of the cell to influence these different things, failures in translation or changes in the level of translation, right? We talked about expression at the level of transcription, true gene expression. We had three things that could happen. What were they? It could go up, it could stay the same or it could go down. Same is true for each of these steps. A change could increase that activity, a change could do nothing to that activity or it could decrease that activity. And if we lose the activity, we lose the outcome of the activity. If we gain the activity to too high of a level, we get too much of that activity inappropriately. The name of the game is regulation. We have to control where and when things happen. And if we deregulate, we can have all sorts of adverse outcomes. So we do think of gene expression as more of a pathway. Now do we know why I split the two? So I could hit the first message on them and bring you two. Guess what, it's more complicated. So each of those steps that we talked about, whether it's architecture, histones, a dentalation, right? Whether it was degradation, translation, folding, localization, phosphorylation, availability through degradation. What's the protein we got rid of to control? We already cycled, didn't we? We built them and broke them to be able to control the kinases. All of those things work together to make you work the way you're supposed to. All of our genes have the potential to function in what we call regulatory networks. What are transcription factors? They control transcription, right? They help to do what to ensure that transcription takes place? They help the RNA polymerase land at the right spot in the right time, right? They improve that relationship. So that high affinity promoters work very, very quickly and lower affinity promoters work when they're supposed to. Right? That's just one part. A transcription factor can control dozens, if not hundreds of genes. And so if we start with one part of a path, we have turning on the transcription factor, making it available, it turning on its downstream, and then each of those downstream pieces having a different activity or perhaps participating in another part of the regulatory network. And so in the end, you can build incredibly complex images and paths for how each of those genes are relating to each other. X turned on Y, Y went on to turn on ABC and D, ABC and D turned on HIJKL, and so on and so forth. And when it comes to this overall, we don't only have transcription factors, we have additional pieces that are all playing together to carry out and control the entire system. And so each of these regulatory networks can be generated and viewed from each specific activity. So you can actually say, I wanna look at migration. What are all the genes and pathways and processes involved? And how do they relate for migration of a cell? And then on top of that, we can look at how that works to create a structure in development. So each of these networks can give us tremendous ideas of what are the key regulatory genes? If I take out X, can I destroy the whole system? And it helps us identify very critical genes in overall processes. And in fact, is one of our most vibrant areas of cancer research, because common players, like this one right here, at the heart of a lot of stuff, that's a great target. Does that make sense? By the way, getting these takes computational analysis. You can't just do this without it. You have to be able to use large scale data sets and use them appropriately. But we can actually find families, binding domains, use binding domains to generate families. We can create an analysis and understanding of how things relate to each other. And every time we look at this, every time we move forward with this, we gain a little bit more insight and a little bit more ability to study this. So at this point, we've moved past just transcription factors to micro-RNAs, bringing a couple of other pieces together. And so now we gotta move down the line. We gotta look at RNA. When it comes to RNA, we don't just leave it alone. We have to play with the RNA. We modify it in more than 100 ways. We modify it chemically, we splice it. But literally, it's actually, I saw a number once where it was like 900 unique modifications can happen. Just chemically, it's over 100. There's some interesting numbers with this, just the sheer diversity we can create from a single RNA molecule. One of the ones that's really fascinating was actually historically referred to as the fifth nucleotide was pseudo-uridine. And so pseudo-uridilation is sort of creating this additional nucleotide from which we can affect things like mRNA stability, the overall ability to translate the molecule, the actual structure of our downstream product. We had talked about this from the perspective of base deamination, right? Now we're talking about chemically modifying uridine changing the potential confirmations that the RNA can take on, enhancing base pairing, reducing base pairing. So here's one chemical, the change that it makes and all the ways that it can influence things all dependent on where it makes that change. So while transcription is our number one way, even just the molecule we transcribe can be altered in this way. And this is regulation. I can choose to chemically alter the RNA to control its half-life or not. This is particularly important for things like tRNAs, for our structural RNA molecules where we can change their shape and stability to help encourage impacts on things like translation. And I just thought this was really fun because there are direct connections to changes in the ability to pseudo-uridylate in terms of cardiovascular health. Modifying RNA is critical to human health. We've talked about all the things we can do to DNA, right? There's a list up there. Tell me what some of them are. We can methylate it. We can acetylate it, right? We can wrap it around a histone. We can use chromatin remodeling complexes, yes? When it comes to those processes, remember some of those are heritable. Some of those changes. Some of the things that we can do actually can be passed on. And so this is what I think is actually a really interesting piece that brings us back to our X and Y chromosomes. The inactivated X appears to have regulatory capacity on autosomes. So we've chosen the chromosome to inactivate and there seems to be some ability for the material on that inactive X to influence the expression of other genes. That is fascinating because this concept of, well, it's just inactive, right? We already knew it wasn't anymore because there are genes that escape inactivation. But now literally the inactive parts seem to play some sort of regulatory role as well. Isn't that fascinating? I think it is. That's why I added it. But also, of course, the Y chromosome can influence autosomes. Nothing exists in a vacuum. We actually don't really have a good understanding of how this process is working. It does appear to be linked to the molecules that contributed to inactivation, like Xist. But it's just a really interesting piece that we have to consider. Nothing you have exists in a vacuum. You do. And of course, there are things that happen to you that you can pass on. Sematic mutation theory, changes in your somatic cells, is now what I'm talking about. You cannot pass on that change that occurred to your skin cell because you spent a lot of time with UV light. But you can pass on if those changes happened dramatically. And you certainly can pass on profile changes that happen systemically. You have an environment. Your epigenetic changes influence that environment. And that, of course, can influence your gametes. Make sense? Some of the best connections to this are connections with things like folic acid, things that can actually change methyltransferase activity. But in addition to that, when it comes to heritable expression profiles, there's a really interesting finding. When you hear the word clone, what do you think? Identical, right? It's a clone. All of our cloning studies, we thought things were gonna be identical. What was an example I gave you where we learned the hard way that wasn't true? When we tried to clone Dolly, and she ended up with too short of telomeres and had an aging condition, right? Because telomere length mattered there. But it turns out, even for things like B cells, which should be incredibly clonal, right? B cells should make the same B cells, should make the same B cell. Turns out that you can end up with what are called clonal identities, where you actually have functional or expressional heterogeneity. Different patterns, even from clones. And those clonal identities actually contribute to behavior. So functional heterogeneity is different than genetic heterogeneity. Genetic heterogeneity implies what? Homogeneity implies what? Let's start there. Everything should be the same. That's what we expect with a clone. Trains should be what? If they're identical. It should be identical. They should contain the same genetic information. When you apply this to situations of populations of cells, if you are a clone, you should be homogeneous. You should have the same genetic pattern. But on top of that, we can add this layer of functionality, right? What the genes are expressing, and to what degree? Clones should still have the same identity at that level if they are truly clones, yes? When you are making daughter cells, what's the goal? It's gotta be the same. So a liver cell should make two liver cells. Should they have different patterns of expression? Not if they're the same type of cell you're trying to create. But it turns out, even in highly clonal situations where everything should be the same clone, we actually get what are called clonal identities where we have different patterns of expression across the whole population of clones. So if I had one cell, and it had expression of A, B, and C, and it made multiple generations of daughter cells, you could actually literally get a population that expresses A, a population that expresses B, and a population that expresses C, even though genetically they're all the same. That is a clonal identity. And that matters in things like cancer. Most tumors can either be thought of as homogeneous or heterogeneous, meaning that all the cells of it should be the same genetic makeup or different. And then you get the level of functional heterogeneity. A breast tumor is heterogeneous. You have multiple cell subtypes. A B cell lymphoma should be homogeneous. They should all be the same B cells because you're just not getting rid of them. What this now tells us is what we previously thought as all being the same is actually not. And it helps explain why a heterogeneous tumor like a breast tumor is actually way more complicated to treat than we previously thought. If even homogeneous material, clonal material, has different identities, then something that's truly different has even more potential for differences. Does this make sense? Complicated picture for treatment. So heritable patterns. And of course, what's influencing these heritable patterns on these clones? Environment. A liver is not the same as the brain. And then we go from that and we move a liver cell to the brain. Well, that's gonna create a different complication, right? So I just want us to appreciate that, yes, things are heritable, but then that pattern of heritability can be influenced by other factors. And clonal identities is a really interesting new implication because it helps to explain how something that should have had universal responses has different responses. Clones should respond the same way to a treatment. And yet we can evade treatment in some conditions. Does this make sense? Clonal identities is a really cool concept. All right, so now we're at the kind of harder part of this that we're gonna do a little bit quickly because our goal is to just sort of summarize using a prokaryotic front. When we have gene regulation at the level of the transcription, we can have inducible versus repressible, right? And it turns out that many of our catabolic enzymes are inducible and many of our anabolic enzymes are repressible. And I think it makes sense because why would you waste energy making something that is only needed when you need to break down something else? Especially if that something else isn't there. Versus keeping something on because you need it as a building block. I literally need it to make all these other things that I make. The operon model is something I've hinted at, but we're essentially looking at that polycystronic concept of linking things, but this is all about the regulation piece. Operators control whether you can be on promoters or where polymerases land to be on. So if I bind material at the operator, it's gonna block the promoter. So operators are a site of repression. And we are gonna focus on lack and trip very briefly. Everything I expect you to know is on this one slide. And it's a lot of words, so we're just gonna sum it up. I am off until I'm needed. I have two major components, but it's all about using lactose. Do I want to be on if there's no lactose? No. So I have a system where I stay off until lactose is present. The reason we will always expect a little bit of activity here is because one of our enzymes is about lactose intake. I can't change my expression if I can't see the lactose. So there's always a little bit of activity here. But glucose, I like more. So if glucose is around, I don't even care if lactose is there. And so we have a system here where we can turn it on, but then also regulate the level of on. So if I've got glucose, lactose, you're just gonna have to wait. But if I do not have glucose and I have a high level of lactose, this is on. Our partner is the opposite because what I am doing is making the ability to make tryptophan. Do I need this? Yes, I do. So I need it on. And so this is negative feedback. When is the one time I don't want tryptophan synthesis running? I'm gonna have enough tryptophan. And so all of our regulation of this is about forming an APO repressor, turning it off when we have enough. And because it's so important that we do that, we actually have multiple steps of regulation. And one of those steps is folding the molecules so we can't transcribe it, known as, or can't translate it, known as attenuation. What's different about our prokaryotic friends? Transcription and translation are coupled. So I can regulate both of those simultaneously. TRNA does that too. If I have enough tryptophan, I'm gonna have plenty of tryptophan TRNA, so I'm gonna shut this down. I'm not gonna want translation. I've got plenty of tryptophan. I don't wanna translate more of the stuff that's gonna make tryptophan. So I realized it was a lot for three slides, and I promise we are gonna practice that with some examples for the level of expectation, but this giant figure is just telling you, trip TRNA can turn things off. And so right here is where my recording for Friday will pick up, and it will do all of the cool prions, which is the next lecture. But then we will actually do a little bit of a review when we hit the ground Monday, okay? So nothing that's in the recording will get ignored for a face-to-face, okay? So please, please, please come to Research Day. Please come and check it out. Any last-second questions, because I went over again by like a minute. I'm sorry, I'm really working on it. Last-second questions? Okay, thank you so much for your time.