Paleoceanography PDF: MECO: A Multiproxy Record of Paleoceanographic Changes (AQA 2014)
Document Details
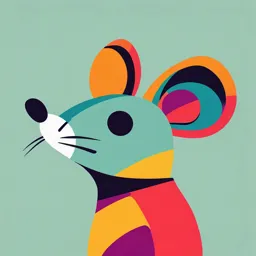
Uploaded by BrotherlyObsidian5111
Université Tunis El Manar Faculté de Médecine de Tunis
2014
AQA
F. Boscolo Galazzo, E. Thomas, M. Pagani, C. Warren, V. Luciani, and L. Giusberti
Tags
Summary
This 2014 AQA past paper details a multiproxy record of paleoceanographic changes during the middle Eocene climatic optimum (MECO) in the southeast Atlantic. The study investigates paleoceanographic, paleoecological, and paleoenvironmental repercussions of this global warming event.
Full Transcript
PUBLICATIONS Paleoceanography RESEARCH ARTICLE The middle Eocene climatic optimum (MECO): 10.1002/2014PA002670 A multiproxy record of paleoceanographic Key Points: Uniform surface to bottom temperature...
PUBLICATIONS Paleoceanography RESEARCH ARTICLE The middle Eocene climatic optimum (MECO): 10.1002/2014PA002670 A multiproxy record of paleoceanographic Key Points: Uniform surface to bottom temperature changes in the southeast Atlantic increase during MECO No water column stratification or (ODP Site 1263, Walvis Ridge) primary productivity changes F. Boscolo Galazzo1, E. Thomas2,3, M. Pagani2, C. Warren2, V. Luciani4, and L. Giusberti1 during MECO Warming may have directly affected 1 Department of Geosciences, University of Padova, Padova, Italy, 2Department of Geology and Geophysics, Yale University, pelagic and benthic ecosystems New Haven, Connecticut, USA, 3Department of Earth and Environmental Sciences, Wesleyan University, Middletown, Connecticut, USA, 4Department of Physics and Earth Sciences, University of Ferrara, Ferrara, Italy Supporting Information: Readme Table S1 Abstract The middle Eocene climatic optimum (MECO, ~40 Ma) was a transient period of global warming that Figure S1 Figure S2 interrupted the secular Cenozoic cooling trend. We investigated the paleoceanographic, paleoenvironmental, and paleoecological repercussions of the MECO in the southeast Atlantic subtropical gyre (Ocean Drilling Correspondence to: Program Site 1263). TEX86 and δ18O records support an ~4°C increase in surface and deepwater temperatures F. Boscolo Galazzo, during the MECO. There is no long-term negative carbon isotope excursion (CIE) associated with the early fl[email protected]; fl[email protected] warming, consistent with other sites, and there is no short-term negative CIE (~50 kyr) during the peak of the MECO, in contrast to what has been observed at some sites. This lack of a CIE during the peak of the MECO at Site 1263 could be due to poor sediment recovery or geographic heterogeneity of the δ13C signal. Citation: Boscolo Galazzo, F., E. Thomas, Benthic and planktic foraminiferal mass accumulation rates markedly declined during MECO, indicating a M. Pagani, C. Warren, V. Luciani, and reduction of planktic foraminiferal production and export productivity. Vertical δ13C gradients do not indicate L. Giusberti (2014), The middle Eocene major changes in water column stratification, and there is no biomarker or micropaleontological evidence climatic optimum (MECO): A multiproxy record of paleoceanographic changes in that hypoxia developed. We suggest that temperature dependency of metabolic rates could explain the the southeast Atlantic (ODP Site 1263, observed decrease in foraminiferal productivity during warming. The kinetics of biochemical reactions Walvis Ridge), Paleoceanography, 29, increase with temperature, more so for heterotrophs than for autotrophs. Steady warming during MECO may 1143–1161, doi:10.1002/2014PA002670. have enhanced heterotroph (i.e., foraminiferal) metabolic rates, so that they required more nutrients. Received 9 MAY 2014 These additional nutrients were not available because of the oligotrophic conditions in the region and Accepted 3 NOV 2014 the lesser response of primary producers to warming. The combination of warming and heterotroph Accepted article online 6 NOV 2014 starvation altered pelagic food webs, increased water column recycling of organic carbon, and decreased Published online 8 DEC 2014 the amount of organic carbon available to the benthos. 1. Introduction The middle Eocene climatic optimum (MECO) is a global warming event which temporarily interrupted the long-term cooling trend initiated at the end of the early Eocene climate optimum (EECO, ~49 Ma). The MECO was first recognized by an ~1‰ negative oxygen isotope excursion in bulk carbonate and benthic foraminiferal tests in Southern Ocean cores [Bohaty and Zachos, 2003], and subsequently identified in the Atlantic Ocean, and central western Tethys [Jovane et al., 2007; Bohaty et al., 2009; Edgar et al., 2010; Spofforth et al., 2010]. Bulk carbonate oxygen isotope records indicate ~4–6°C warming (assuming ice-free conditions), with a gradual onset and brief peak temperatures, followed by a rapid return to preevent temperatures. The available benthic foraminiferal oxygen isotope records, mostly from the Southern Ocean, are similar. High-resolution stratigraphic analysis of cores from Site 1260 (tropical west Atlantic) indicates that the warming event lasted between 400 and 500 kyr, with a gradual onset starting at ~40.45–40.5 Ma and peak warming by 40.05 + 0.02 Ma [Bohaty et al., 2009; Westerhold and Röhl, 2013]. The MECO occurred during a very long eccentricity cycle minimum, thus at relatively attenuated seasonality at lower latitudes [Westerhold and Röhl, 2013]. Its duration and pattern of warming differentiate the MECO from upper Paleocene-lower Eocene hyperthermal events [Bohaty et al., 2009], which are characterized by more symmetrical patterns of warming and cooling that lasted a few tens of thousands of years and generally occurred during eccentricity maxima [Thomas and Zachos, 2000; Cramer et al., 2003; Bowen and Zachos, 2010; Galeotti et al., 2010; Stap et al., 2010; Zachos et al., 2010]. Warming during these hyperthermals was coeval BOSCOLO GALAZZO ET AL. ©2014. American Geophysical Union. All Rights Reserved. 1143 19449186, 2014, 12, Downloaded from https://agupubs.onlinelibrary.wiley.com/doi/10.1002/2014PA002670 by Tunisia Hinari NPL, Wiley Online Library on [08/12/2024]. See the Terms and Conditions (https://onlinelibrary.wiley.com/terms-and-conditions) on Wiley Online Library for rules of use; OA articles are governed by the applicable Creative Commons License Paleoceanography 10.1002/2014PA002670 40 Ma Reconstruction Figure 1. Drill sites and sections on land where the MECO has been described. Approximate positions at 40 Ma are plotted on a paleogeographic reconstruction from the Ocean Drilling Stratigraphic Network (GEOMAR, Kiel, Germany). (top) Sites where the negative CIE is well expressed are labeled with a solid triangle, sites with a doubtful CIE are labeled with an open triangle, and sites where no data are available are labeled with an open circle with a cross. (bottom) Sites with inferred increased productivity during the MECO are labeled with solid circles, sites with inferred decreased or unchanged pro- ductivity are labeled with open circles, and sites where no data are available are labeled with open circles with a cross. with a negative carbon isotope excursion (CIE) and carbonate dissolution, indicative of ocean acidification [Zachos et al., 2005; Lunt et al., 2011; Leon-Rodriguez and Dickens, 2010; McInerney and Wing, 2011; Hoenisch et al., 2012; Penman et al., 2014], caused by a relatively rapid release of 13C-depleted carbon [Zachos et al., 2005, 2008; McInerney and Wing, 2011; Hoenisch et al., 2012]. The MECO has been described as a global carbon cycle perturbation [Bijl et al., 2010], but the lack of a CIE contemporaneous with warming and the duration of the climate anomaly suggest that the event was more complex than the earlier hyperthermals [Sluijs et al., 2013]. There is no evidence for the release of isotopically light carbon coupled to MECO warming. Marine carbonate δ13C records show considerable geographic and bathymetric variability but are usually characterized by rising rather than declining δ13C values during the initial gradual warming [Bohaty and Zachos, 2003; Bohaty et al., 2009; Borelli et al., 2014]. A transient, ~0.5‰ negative CIE (~50 kyr) during peak warming occurs at some but not all sites (Figure 1) [Bohaty et al., 2009; Edgar et al., 2010; Spofforth et al., 2010]. Coeval with the MECO was a widespread, ~1 Myr long decrease in carbonate mass accumulation rate at water depths greater than 3000 m in the Indian and SE Atlantic Oceans [Bohaty et al., 2009]. In the equatorial Pacific, carbonate mass accumulation rates declined at sites at depths greater than 2000 m [Hancock and Dickens, 2005; Bohaty et al., 2009]. The carbonate compensation depth (CCD) shoaled from ~4100 to ~3200 m depth [Pälike et al., 2012], and the preservation of nannofossils decreased [Toffanin et al., BOSCOLO GALAZZO ET AL. ©2014. American Geophysical Union. All Rights Reserved. 1144 19449186, 2014, 12, Downloaded from https://agupubs.onlinelibrary.wiley.com/doi/10.1002/2014PA002670 by Tunisia Hinari NPL, Wiley Online Library on [08/12/2024]. See the Terms and Conditions (https://onlinelibrary.wiley.com/terms-and-conditions) on Wiley Online Library for rules of use; OA articles are governed by the applicable Creative Commons License Paleoceanography 10.1002/2014PA002670 2013], as the abundance of benthic foraminifera [Takata et al., 2013]. The MECO-associated CCD rise occurred within the 2 Myr interval between carbonate accumulation events CAE-3 and CAE-4 [Lyle et al., 2005, 2008; Pälike et al., 2012] and was one of several, similar CCD events superimposed on a longer-term trend of CCD deepening in the Pacific Ocean [Pälike et al., 2012]. Only a few studies have focused on the paleoenvironmental and paleobiotic consequences of the MECO. The event led to a significant crisis of muricate planktic foraminifera (acarininids and morozovelloids [Luciani et al., 2010; Edgar et al., 2013]) and temporary loss of photosymbionts (bleaching) in Acarinina [Edgar et al., 2013], possibly caused by the warming itself. Integrated studies of various microfossil and geochemical proxies in a western Tethys section deposited at middle-bathyal paleodepth [Spofforth et al., 2010; Luciani et al., 2010; Toffanin et al., 2011; Boscolo Galazzo et al., 2013] record higher export productivity during the MECO peak and directly afterward [Boscolo Galazzo et al., 2013]. At Site 1051 (NW Atlantic), diatoms and silicoflagellates increased in abundance during MECO initial warming, then decreased at peak MECO, while benthic foraminiferal data indicate an increase in delivery of organic matter to the seafloor [Moebius et al., 2013; Witkowski et al., 2014]. Siliceous phytoplankton also peaked in abundance at Southern Ocean Sites 748 and 749 (Indian Ocean sector [Witkowski et al., 2012]), with increased primary productivity supported by nannofossil data [Villa et al., 2014]. At Site 738 (Kerguelen Plateau), benthic foraminiferal assemblage data combined with a cerium anomaly suggest decreased bottom water oxygenation during peak warming, whereas productivity and export productivity increased during the entire event [Moebius et al., 2014]. Calcareous nannoplankton assemblages indicate eutrophic conditions at Southern Ocean Site 738 [Villa et al., 2014]. In contrast, dinocyst assemblages at Ocean Drilling Program (ODP) Site 1172, a marginal marine shelf area at southern high latitudes, indicate lower productivity [Bijl et al., 2010], consistent with interpretation of calcareous nannofossil assemblages at Site 702 (subpolar South Atlantic) during peak warming [Pea, 2011]. Pacific CAEs are argued to have been high-productivity events, as confirmed by tentative benthic foraminiferal data indicating increased delivery of organic matter to the seafloor [Takata et al., 2013], with the MECO reflecting a relatively low productivity interval at equatorial Pacific sites [Lyle et al., 2005, 2008]. Thus, there is clear evidence for substantial geographic differences in oceanic productivity during the event (Figure 1). For this study, we generated stable oxygen and carbon isotopic records from bulk carbonate and benthic and planktic foraminifera at ODP Site 1263 on the Walvis Ridge in the midlatitude southeastern Atlantic Ocean [Zachos et al., 2004; Bohaty et al., 2009], in order to investigate the expression of the MECO throughout the water column. Sea surface temperature (SST) changes were estimated using the TEX86 proxy, because planktic foraminifera were recrystallized, compromising oxygen isotope values as commonly observed [Pearson et al., 2001; Sexton et al., 2006]. Carbon isotope records were used to infer changes in ocean stratification and compared with benthic foraminiferal accumulation rate (BFAR), a proxy for primary productivity in the absence of changes in delivery of organic matter to the seafloor [Herguera and Berger, 1991]. This study is one of the the first multiproxy investigations of the MECO in an open-ocean setting and aims to improve our understanding of this climatic perturbation and its paleoceanographic and paleoecological repercussions. 2. Location and Setting ODP Site 1263 (28°53′S, 2°78′E, modern water depth 2717 m; ~800 km off the coast of Africa; Figure 1) was drilled during ODP Leg 208 on the north facing flank of Walvis Ridge (SE Atlantic Ocean [Zachos et al., 2004]). Site 1263 lies beneath the eastern part of the central subtropical gyre, outside the eastern boundary current with its associated high productivity. The seafloor at Site 1263 presently is bathed by Antarctic Intermediate Water, with North Atlantic Deep Water at slightly greater depths [Klevenz et al., 2008]. In the middle Eocene, Site 1263 was at a paleodepth of about 2000 m [Zachos et al., 2004] and likely bathed by a southern sourced, intermediate to deepwater mass [Via and Thomas, 2006; Thomas and Via, 2007; Stap et al., 2010; Borelli et al., 2014]. Eocene sediments are nannofossil ooze, foraminifer-bearing nannofossil ooze, clay-bearing nannofossil ooze, and chalky nannofossil ooze, with a low concentration of total organic carbon (0.86–0.00 wt %; mean = 0.09 wt % [Zachos et al., 2004]). Sediments vary in color between light gray and gray at decimeter scale and contain white, 1–4 cm “blebs” surrounded by dark haloes containing fine-grained, black to brown oxides [Zachos et al., 2004], which may be cross sections of burrows. Site 1263 has a complete and relatively expanded record across the MECO, with an average linear sedimentation rate for the studied interval of 0.74 cm/kyr [Bohaty et al., 2009] and well-constrained nannofossil biostratigraphy [Fornaciari et al., 2010]. BOSCOLO GALAZZO ET AL. ©2014. American Geophysical Union. All Rights Reserved. 1145 19449186, 2014, 12, Downloaded from https://agupubs.onlinelibrary.wiley.com/doi/10.1002/2014PA002670 by Tunisia Hinari NPL, Wiley Online Library on [08/12/2024]. See the Terms and Conditions (https://onlinelibrary.wiley.com/terms-and-conditions) on Wiley Online Library for rules of use; OA articles are governed by the applicable Creative Commons License Paleoceanography 10.1002/2014PA002670 3. Methods We generated bulk CaCO3 stable isotopes and TEX86 data on 46 samples (Table S1 in the supporting information). The bulk isotope record has a 20 cm sample resolution within the MECO, 40 cm above and below. An additional set of 34 samples spaced at 40 cm was used for foraminiferal stable isotope analyses, accumulation rate, and planktic/benthic foraminifer calculations (Table S1 in the supporting information). We used the relatively simple age model adopted by Bohaty et al. , based on magnetobiostratigraphy and correlations to other sites using stable isotope records. We report data as a function of depth (meter composite depth (mcd)) and age [Pälike et al., 2006], at a resolution of ~25 kyr for the bulk record and ~50 kyr for foraminiferal records. 3.1. Stable Isotope Measurements Samples were air dried and weighed, disaggregated in deionized water, and washed through sieves with mesh sizes of ≥38, ≥63, and ≥500 μm. The size fraction larger than 63 μm was weighed and split into two equal parts with a precision microsplitter. Benthic foraminiferal stable isotope records (δ13C and δ18O) were generated for the epifaunal species Nuttallides truempyi [Thomas and Shackleton, 1996; Katz et al., 2003], following taxonomy by van Morkhoven et al.. Planktic foraminiferal stable isotope records (δ13C and δ18O) were generated on mixed species of the surface-dwelling, photosymbiont-bearing genus Acarinina [Pearson et al., 2006]. The species Acarinina topilensis and A. praetopilensis were selected to limit interspecific variability, but this was not always possible due to the decrease in abundance of muricate planktic foraminifera during peak warming (139.4–137.4 mcd) and ~3–4 m higher. Benthic and Acarinina stable isotope analyses were carried out on specimens from the size fraction between 250 and 180 μm in samples between 145 and 132 meter composite depth (mcd). We also generated a stable isotope record at 40 cm sample resolution for the mixed layer genus Globigerinatheka [e.g., Pearson et al., 2006] in the ≥500 μm size fraction for the 145–136 mcd interval. Bulk CaCO3 stable isotopes were measured for the interval between 147 and 132 mcd. Bulk and foraminiferal samples were analyzed for stable oxygen and carbon isotopes by acid digestion using an individual vial acid drop ThemoScientific Kiel IV carbonate device interfaced to Thermo Scientific MAT-253 dual-inlet isotope ratio mass spectrometer (IRMS) at the University of California, Santa Cruz. Samples were reacted at 75°C in orthophosphoric acid (specific gravity = 1.92 g/cm3) to generate carbon dioxide and water. During this procedure, water was cryogenically removed from CO2, and noncondensable gases are pumped away, prior to the introduction of the purified CO2 into the IRMS. A calibrated, in-house standard of Carrara Marble was used to correct analytical offsets. Four NBS-18 limestone standards were used in conjunction with Carrara Marble to correct for instrument-specific source ionization effects. NBS-19 standards were used to monitor quality control and long-term performance. Carrara Marble has been extensively calibrated against National Institute of Standards and Technology Standard Reference Materials (NBS-19, NBS-18, and LSVEC) and as part of intercomparison studies with other stable isotope laboratories. Sets of standards were run before and after running the samples; analytical precision is estimated at 0.04‰ for δ13C and 0.06‰ for δ18O. Corrected delta values are expressed relative to international standards Vienna Peedee belemnite for δ13C and δ18O. To compare our records with those in Bohaty et al. , oxygen isotope values were converted to paleotemperatures using the equation of Shackleton , assuming a global mean δ18O for seawater (δ18Osw) of 1.0‰, representing an ice-free world [Zachos et al., 1994; Huber et al., 2003; Burgess et al., 2008; Bohaty et al., 2009], although the presence of small continental ice sheets on Antarctica cannot be ruled out [Tripati et al., 2005; Edgar et al., 2007; Dawber and Tripati, 2011; Bohaty et al., 2009]. 3.2. Tetraether Lipid Analysis Samples were processed and analyzed for glycerol dialkyl glycerol tetraether (GDGT) lipids in the Organic Biogeochemistry and Paleoclimatology Laboratory at Yale University following Schouten et al. [2002, 2013]. Compounds were extracted from freeze-dried sediment samples with a soxhlet extractor using a 2:1 dichloromethane:methanol solvent mixture. The lipid extracts were then separated into apolar, ketone, and polar fractions via silica gel column chromatography. GDGTs were further purified via alumina chromatography and filtered through 0.45 μm glass fiber filters. Quantification of GDGTs was conducted BOSCOLO GALAZZO ET AL. ©2014. American Geophysical Union. All Rights Reserved. 1146 19449186, 2014, 12, Downloaded from https://agupubs.onlinelibrary.wiley.com/doi/10.1002/2014PA002670 by Tunisia Hinari NPL, Wiley Online Library on [08/12/2024]. See the Terms and Conditions (https://onlinelibrary.wiley.com/terms-and-conditions) on Wiley Online Library for rules of use; OA articles are governed by the applicable Creative Commons License Paleoceanography 10.1002/2014PA002670 using high-performance liquid chromatography–atmospheric pressure chemical ionization mass spectrometry (HPLC-APCI-MS; Agilent1200 series liquid chromatograph and Agilent 6130 series single quadrupole mass spectrometer), following Schouten et al.. A Prevail Cyano column was used with 99:1 hexane:isopropanol as eluent. The solvent gradient was 100% hexane/isopropanol (99:1, vol/vol; 5 min), then increasing the amount of hexane/isopropanol at a ratio of 90:10 vol/vol (0% at 5 min to 7.4% at 40 min; held 10 min). Samples were scanned in selected ion monitoring mode. Peak integration was carried out using Agilent Chemstation. Analytical precision is estimated at ±0.01 TEX86 units. TEX86 values were calculated using the equation of Schouten et al. : TEX86 ¼ ½GDGT2 þ GDGT3 þ cren′=½GDGT1 þ GDGT2 þ GDGT3 þ cren′ (1) where GDGTs 1–3 indicate compounds containing 1–3 cyclopentyl moieties, and cren′ denotes the regioisomer of crenarchaeol. Several TEX86 temperature calibrations have been derived [e.g., Pearson and Ingalls, 2013]. Kim et al. recommend the use of the TEXH86 for SSTs >15°C, implying that it would be appropriate for the middle Eocene subtropics, but Hollis et al. show the best fit among Eocene low-latitude SSTs derived from carbonate proxies and >0.85 TEX86 values with Liu et al.’s calibration. Accordingly, we apply both the high-temperature logarithmic calibration of Kim et al. : SST ¼ 68:4½ logðTEX86 Þ þ 38:6 (2) and the nonlinear calibration of Liu et al. : SST ¼ 50:475 16:332ð1=TEX86 Þ (3) to estimate SST change during the MECO. We also applied the Bayesian calibration model (Bayesian Spatially Varying Regression) “deep time” version developed by Tierney and Tingley. Relative to prior TEX86 calibrations, the Bayesian model propagates calibration uncertainty and allows model parameters to vary spatially. The Branched and Isoprenoid Tetraethers Index, a proxy for terrestrial organic matter input, was calculated following Hopmans et al. in order to evaluate the influence of terrestrial organic matter input that arguably biases TEX86 temperature estimates: BIT ¼ ½I þ II þ III=½I þ II þ III þ IV (4) where compounds I, II, and III represent the branched GDGTs derived from soil bacteria and compound IV is crenarchaeol, an isoprenoidal GDGT primarily derived from marine archaea, a compound distinct from the crenarchaeol regioisomer used in the calculation of TEX86. 3.3. Foraminiferal and Fine Fraction Accumulation Rates The benthic foraminiferal accumulation rate (BFAR) was calculated from the numbers in representative splits of the size fraction ≥63 μm containing ≥400 specimens, corrected to the weight of bulk sediment. The BFAR is a proxy for total organic matter flux reaching the seafloor [e.g., Gooday, 2003; Jorissen et al., 2007], because benthic foraminifera rely on the organic matter produced in the surface and exported down in the water column. Organic flux reaching the seafloor is a function of primary productivity and remineralization of organic matter in the water column [e.g., Henson et al., 2011; Arndt et al., 2013; Ma et al., 2014]. We calculated the coarse fraction as the weight ratio of the dry ≥63 μm size fraction to the bulk dry sediment weight. The fine fraction was calculated as the weight ratio of the bulk dry sediment minus the coarse fraction weight to the bulk dry sediment. Coarse and fine fraction accumulation rates (CFAR and FFAR) were calculated using linear sedimentation rates and dry bulk density. Linear sedimentation rates were derived using the age model of Bohaty et al.. Dry bulk density was estimated by extrapolating the density values for the studied interval [Zachos et al., 2004], which show little variability. The benthic foraminiferal accumulation rate (BFAR) was calculated following Herguera and Berger : BFAR number of specimens cm2 kyr1 ¼ N=g * LSR * DBD (5) where N/g is the number of benthic foraminifera per gram dry bulk sediment, LSR is the linear sedimentation rate (cm/kyr), and DBD is the dry bulk density (g/cm3). BOSCOLO GALAZZO ET AL. ©2014. American Geophysical Union. All Rights Reserved. 1147 19449186, 2014, 12, Downloaded from https://agupubs.onlinelibrary.wiley.com/doi/10.1002/2014PA002670 by Tunisia Hinari NPL, Wiley Online Library on [08/12/2024]. See the Terms and Conditions (https://onlinelibrary.wiley.com/terms-and-conditions) on Wiley Online Library for rules of use; OA articles are governed by the applicable Creative Commons License Paleoceanography 10.1002/2014PA002670 18 Figure 2. (top) ODP Site 1263 TEX86 and δ O data plotted versus meter composite depth. The black line in the TEX86 SST plot is the three-point running mean. (bottom) TEX86 , BIT indices and cren/g bulk plotted versus meter composite depth. The black line in the TEX86 SST plot is the three-point running mean. Cren/g bulk is the abundance of the biomarker crenarchaeol from peak area integrations normalized to the weight of bulk sediment. The area shaded in gray highlights the entire MECO interval, with light gray indicating the peak of the MECO. Biostratigraphy after Fornaciari et al. following (1) calcareous nannofossil zonation of Fornaciari et al. and (2) nannofossil standard zonation of Okada and Bukry. Age model after Bohaty et al.. We used CFAR as an approximation of the planktic foraminiferal accumulation rate, because this fraction dominantly consists of planktic foraminifera in pelagic sediments not significantly affected by dissolution [Diester-Haass, 1995]. In typical oceanic calcareous oozes dominated by calcareous nannofossils, FFAR can, with some caution, be considered indicative of the calcareous nannofossil accumulation rate. We calculated the planktic/benthic foraminiferal value (P/(P + B)%, briefly P/B), an indicator of carbonate dissolution [Berger and Diester-Haass, 1988] expressed as the proportion of planktic specimens in the total foraminiferal assemblage (≥63 μm fraction), for each sample across the MECO and in some samples below and above, counting a consistent number of planktic foraminiferal tests (600–630). 4. Results 4.1. Oxygen Isotope Records The negative δ18O excursion typical for the MECO is present in the benthic, bulk, and Globigerinatheka records. In contrast, the Acarinina record does not show a δ18O excursion, but above 136.8 mcd, values slightly increase to the top of the studied section (Figure 2). Globigerinatheka δ18O values decrease upward from the base (145 mcd). The lowest Globigerinatheka δ18O values occur at 139.6–138.4 mcd for a total excursion of 0.9‰ (~4°C), from 0.4 to 0.5‰, and recover to preexcursion values within 40 cm (138.4–138 mcd) corresponding to ~50 kyr (Figure 2). BOSCOLO GALAZZO ET AL. ©2014. American Geophysical Union. All Rights Reserved. 1148 19449186, 2014, 12, Downloaded from https://agupubs.onlinelibrary.wiley.com/doi/10.1002/2014PA002670 by Tunisia Hinari NPL, Wiley Online Library on [08/12/2024]. See the Terms and Conditions (https://onlinelibrary.wiley.com/terms-and-conditions) on Wiley Online Library for rules of use; OA articles are governed by the applicable Creative Commons License Paleoceanography 10.1002/2014PA002670 18 18 Figure 3. (top) Site 1263 bulk carbonate δ O data plotted versus numerical age. (a) Bulk δ O data generated in the present study combined with bulk data in Bohaty et al.. (b) Bulk isotope data from Bohaty et al.. (c) Bulk 18 isotope data generated in this study. (bottom) Compilation of benthic δ O records for the MECO plotted versus numerical age and compared with bulk/fine fraction records from the same sites (thinner lines). Sites 738 and 748 data are from Bohaty et al. , Site 1051 data are from Bohaty et al. , and Edgar et al. aligned on the Bohaty et al.’s age model. Biostratigraphy after Fornaciari et al. following (1) calcareous nannofossils zonation of Fornaciari et al. and (2) nannofossil standard zonation of Okada and Bukry. Age model after Bohaty et al.. Our bulk δ18O data closely match those from Bohaty et al. (Figure 3), with stable values around 0.5‰ from the base (147 mcd; 41.2 Ma) up to ~142.60 mcd. Values gradually decrease up to ~139 mcd, where a sharp negative ~0.4‰ δ18O shift is recorded. Minimum values occur at 138.7–138.1 mcd, and the overall excursion is 1‰ (corresponding to 4°C; Figure 4). The δ18O values rapidly recover, reaching preevent values at 137.4 mcd, 70 cm (~100 kyr) above the minimum value. Between 137.3 and 136 mcd, bulk δ18O values start to gradually increase up to the top of the studied interval (Figures 2–4). Nuttallides truempyi δ18O values are stable, varying around 0.6‰ from the base of the record up to ~140.8 mcd, and then markedly decrease with a minimum between 138.4 and 138 mcd, and reaching a total excursion of 0.9‰ (Figures 2 and 3). The recovery starts at 138 mcd, reaching preexcursion values by ~136 mcd, 200 cm (270 kyr) above peak MECO (Figures 2, 3, and 4). In total, a deepwater temperature increase of 4°C is estimated from ~9.5 to ~13.5°C (Figure 4). A similar decrease in δ18O values is recorded in benthic, bulk, and Globigerinatheka records (Figures 2 and 4), but the main phases of the MECO (i.e., onset, peak warming, and termination) occur at slightly different depths. Changes in the Globigerinatheka record occur lowest, followed by up-section of those in the bulk and the N. truempyi record (Figure 2). In addition, the MECO and peak warming occur over a thinner core section BOSCOLO GALAZZO ET AL. ©2014. American Geophysical Union. All Rights Reserved. 1149 19449186, 2014, 12, Downloaded from https://agupubs.onlinelibrary.wiley.com/doi/10.1002/2014PA002670 by Tunisia Hinari NPL, Wiley Online Library on [08/12/2024]. See the Terms and Conditions (https://onlinelibrary.wiley.com/terms-and-conditions) on Wiley Online Library for rules of use; OA articles are governed by the applicable Creative Commons License Paleoceanography 10.1002/2014PA002670 18 Figure 4. ODP Site 1263 oxygen and carbon isotope records plotted versus meter composite depth. The δ O axis is inverted to give a positive temperature increase in the upward direction. Biostratigraphy after Fornaciari et al. following (1) calcareous nannofossil zonation of Fornaciari et al. and (2) nannofossil standard zonation of Okada and Bukry. Age model after Bohaty et al.. (i.e., equivalent to a shorter duration) in the benthic and bulk carbonates than in the Globigerinatheka record. Recovery to preexcursion values is sharp in the Globigerinatheka record, whereas bulk and N. truempyi records show a more gradual recovery (Figure 2). The shape of the benthic δ18O excursion appears more symmetrical than those in planktic and bulk-carbonate records (Figure 2). Below the MECO, values and patterns in bulk and benthic foraminiferal δ18O are very similar (Figures 2–4), but bulk values above MECO are higher than in N. truempyi. Globigerinatheka yields generally slightly lower values than both N. truempyi and bulk (Figures 2 and 4). Globigerinatheka is a symbiont-bearing mixed layer dweller [Pearson et al., 1993; Pearson et al., 2001; Wade and Kroon, 2002], but stable isotope values commonly indicate calcification in deeper waters [Wade, 2004; Sexton et al., 2006; Premoli Silva et al., 2006; Burgess et al., 2008, present study] (Figures 2 and 4), suggesting that the genus had late-stage calcification, with CaCO3 crusts forming deeper in the water column [Pearson et al., 2001; Premoli Silva et al., 2006]. Globigerinatheka isotope records thus reflect conditions between the mixed layer and the thermocline [Boersma et al., 1987; Wade, 2004; Sexton et al., 2006], as also indicated by boron isotope data [Pearson and Palmer, 2000]. Acarinina, a photosymbiont-bearing genus calcifying within the mixed layer, has somewhat more negative δ18O values above and below the MECO interval, but across MECO, its values overlap with the Globigerinatheka, bulk, and N. truempyi records because of the lack of an excursion (Figures 2 and 4). 4.2. TEX86 Record and BIT Index Isoprenoidal GDGTs containing cyclopentane rings are in low abundance over the studied interval. Despite the analysis of large (50 cm3) samples, only 29 of the 46 samples analyzed had concentrations above the detection limit. Concentrations in samples between 147 and 143 mcd and from 138 mcd to the top of the studied interval were generally below detection, but the abundance of marine isoprenoidal GDGTs was somewhat higher between 142 and 138 mcd, coincident with the MECO (Figure 2). Using the high-temperature calibration of Kim et al. , TEX86 values of ~0.76 between 147 and 145.22 mcd imply a mean surface temperature of ~30.5°C (Figure 2). A gradual up-section increase in TEX86 values by 0.2 occurs between ~144.8 mcd and 142 mcd, implying warming from 30.5 to 33°C (Figure 2). From 141.5 mcd upward, TEX86-SSTs show an abrupt increase of 1°C. This interval displays strong fluctuations with a large decrease (from 0.85 to 0.7) between ~140 and 138.7 mcd and a rapid recovery to the highest temperature recorded (~34.5°C) between 138.7 and 138.3 mcd, followed by a rapid decrease to 29°C (Figure 2). BOSCOLO GALAZZO ET AL. ©2014. American Geophysical Union. All Rights Reserved. 1150 19449186, 2014, 12, Downloaded from https://agupubs.onlinelibrary.wiley.com/doi/10.1002/2014PA002670 by Tunisia Hinari NPL, Wiley Online Library on [08/12/2024]. See the Terms and Conditions (https://onlinelibrary.wiley.com/terms-and-conditions) on Wiley Online Library for rules of use; OA articles are governed by the applicable Creative Commons License Paleoceanography 10.1002/2014PA002670 Our data indicate a maximum SST excursion of 4.5°C (Figure 2) using TEXH86. The use of the calibration of Liu et al. results in the same trend, but lower overall temperatures and less warming (3°C, from background 29 to 32°C at peak warmth), although this temperature difference is within the calibration error of our data [Liu et al., 2009; Kim et al., 2010]. SSTs derived from the Bayesian regression model as well as the shape and magnitude of MECO temperature excursion are very close to those derived from the other two calibrations (range of uncertainty is ±2–3°C). TEX86-derived SSTs are much higher than those estimated from planktic δ18O values (~14°C), although the magnitude of the temperature excursion is similar. Surface temperatures are considerably higher than those reported for Eocene Thermal Maximum 2 from a single TEX86 data point integrated over 35 cm of sediment at Site 1263 (~25°C [Stap et al., 2010], using both Kim et al.’s and Liu et al.’s calibrations). Branched and isoprenoid tetraether (BIT) indices are very high in all samples, with a maximum of 0.8 (mean 0.55; Figure 2). Such high values are usually found in coastal environments close to fresh water influx carrying terrestrially derived organic matter [Hopmans et al., 2004; Smith et al., 2012]. A BIT index >0.3 has been used to imply that TEX86 temperature estimates are compromised by the presence of soil-derived isoprenoidal GDGTs [Weijers et al., 2006]. However, Site 1263 is in an open-ocean setting ~800 km from land [Moore et al., 1984], and therefore, it appears unrealistic that high BIT values represent a substantial amount of riverine-delivered organic matter. Aeolian dust can carry soil-derived GDGTs to open-ocean areas, but odd numbered long-chain n-alkanes are usually more abundant than GDGTs in dust samples [Fietz et al., 2013]. Long chain n-alkanes with odd- over-even carbon predominance, indicative of a terrestrial higher plant origin, are below detection limit in all of our samples. In addition, BIT index values and TEX86 are not significantly correlated (R2 = 0.1763; Figure S1 in the supporting information) [Huguet et al., 2009]. High BIT indices could possibly be explained by a greater preservation potential of branched GDGTs. In oxidized marine sediments, isoprenoidal GDGTs have lower preservation efficiency than terrestrially derived branched GDGTs, and their preferential degradation increases BIT indices without affecting TEX86 values [Huguet et al., 2009; Lengger et al., 2013]. Alternatively, in situ production of branched GDGTs in marine sediments is possible [Peterse et al., 2009; Zhu et al., 2011; Fietz et al., 2012; Hu et al., 2012; Lengger et al., 2013]. Accordingly, we suggest that the locality of Site 1263 and the lack of higher plant leaf waxes argue against the influence of terrestrially derived GDGTs on our TEX86-derived temperatures and assume that TEX86 SST estimates are robust, particularly the SST trends. 4.3. Carbon Isotope Records The Acarinina δ13C record, similar to its δ18O record, shows variability without a trend (Figure 4). Globigerinatheka δ13C values vary (0.2‰) from the start of the event to ~142 mcd around an average value of 2.5‰, then increase from ~141.4 mcd upward until ~139.5 mcd, with peak values of 3‰, coinciding with the lowest δ18O values. The δ13C values decrease above this level but remain higher than the preevent values (Figure 4). Our bulk δ13C data closely match with the data of Bohaty et al. (Figure 5). Bulk δ13C values show minor (~0.2‰) variability around a mean value of 2.2‰ from the onset of the MECO to 137.8 mcd, coinciding with minimum δ18O values (Figures 4 and 5). At 137.8 mcd, bulk δ13C values increase up-section by about 0.5‰, then remain stable throughout the studied interval (Figures 4). Benthic δ13C values are ~1.5‰ lower than bulk δ13C values and increase from ~144 to ~141 mcd, then remain stable, with a small decline at ~138 mcd, the top of the MECO interval with the most negative δ18O values. The δ13C values increase up-section and then remain stable up to the top of the studied interval, fluctuating around 1.2 ‰ (Figures 4 and 5). Overall, δ13C records of Globigerinatheka, benthic, and bulk gradually become more positive upward in the section (Figures 4 and 5; see also Figure 5 in Borelli et al. ). The δ13C increase coincides with the negative oxygen isotope excursion in benthic and Globigerinatheka records, whereas the increase starts above the MECO interval in the bulk record. In contrast to other sites (e.g., Southern Ocean Sites 738 and 748 [Bohaty et al., 2009]) (Figure 5), a brief negative CIE coinciding with peak warming (minimum in the δ18O records) is lacking at Site 1263, although bulk and benthic records show a minor decrease in δ13C values at the top of the MECO interval (~138 mcd; Figures 4 and 5). BOSCOLO GALAZZO ET AL. ©2014. American Geophysical Union. All Rights Reserved. 1151 19449186, 2014, 12, Downloaded from https://agupubs.onlinelibrary.wiley.com/doi/10.1002/2014PA002670 by Tunisia Hinari NPL, Wiley Online Library on [08/12/2024]. See the Terms and Conditions (https://onlinelibrary.wiley.com/terms-and-conditions) on Wiley Online Library for rules of use; OA articles are governed by the applicable Creative Commons License Paleoceanography 10.1002/2014PA002670 13 13 Figure 5. (top) Site 1263 bulk carbonate δ C data plotted versus numerical age. (a) Bulk δ C data generated in this study 13 combined with bulk δ C data in Bohaty et al.. (b) Bulk isotope data from Bohaty et al.. (c) Bulk isotope 13 data generated in this study. (bottom) Compilation of benthic δ C data for the MECO interval plotted versus numerical age and compared with bulk/fine fraction records from the same sites (thinner lines). Sites 738 and 748 data are from Bohaty et al. , Site 1051 data are from [Bohaty et al., 2009], and Edgar et al. aligned on Bohaty et al. age model. Biostratigraphy after Fornaciari et al. following (1) calcareous nannofossils zonation of Fornaciari et al. and (2) nannofossil standard zonation of Okada and Bukry. Age model after Bohaty et al.. 4.4. Foraminiferal and Fine Fraction Accumulation Rates Benthic foraminiferal (BFAR) and coarse fraction (CFAR; i.e., planktic foraminifera) accumulation rates covary throughout the studied interval. From the base of the studied interval, both CFAR and BFAR increase, reaching peak values at about 142–141 mcd. Values then decrease up-section, reaching a minimum at about 139 mcd, the interval of peak MECO warming. Above this interval, values remain relatively stable (Figure 6). Fine fraction accumulation rate (FFAR) values vary throughout the studied interval without a discernible trend (Figure 6). The P/B remains constant throughout the studied interval (mean 97%), with fluctuations across MECO in the range of 1–2% (Figure 6). 5. Discussion 5.1. Temperature Anomaly The planktic foraminiferal assemblage at Site 1263 appears moderately well preserved but with tests exhibiting “frosty” preservation [Sexton et al., 2006]. Frosty foraminifera are known to be affected by diagenesis, and their δ18O values reflect partial recrystallization (as defined, e.g., in Pearson et al. , Sexton et al. , and BOSCOLO GALAZZO ET AL. ©2014. American Geophysical Union. All Rights Reserved. 1152 19449186, 2014, 12, Downloaded from https://agupubs.onlinelibrary.wiley.com/doi/10.1002/2014PA002670 by Tunisia Hinari NPL, Wiley Online Library on [08/12/2024]. See the Terms and Conditions (https://onlinelibrary.wiley.com/terms-and-conditions) on Wiley Online Library for rules of use; OA articles are governed by the applicable Creative Commons License Paleoceanography 10.1002/2014PA002670 18 Figure 6. ODP Site 1263 bulk carbonate percentage (bold line) and δ O (thin line), planktic/benthic foraminiferal value (P/B), cren/g bulk, benthic foraminiferal, coarse fraction, and fine fraction accumulation rates (BFAR, CFAR, and FFAR) plotted versus meter composite depth. Cren/g bulk is the abundance of the biomarker crenarchaeol from peak area integrations normalized to the weight of bulk sediment. CaCO3% from Bohaty et al.. Biostratigraphy after Fornaciari et al. following (1) calcareous nannofossil zonation of Fornaciari et al. and (2) nannofossil standard zonation of Okada and Bukry. Age model after Bohaty et al.. Pearson ) at seafloor temperatures, which are much lower than those in the surface waters where the foraminifera calcified [e.g., Schrag et al., 1995; Pearson et al., 2001; Stap et al., 2010; Kozdon et al., 2011]. Carbonate oozes at Walvis Ridge consist primarily of calcareous nannofossils, as shown by the very high FFAR relative to CFAR (Figure 6). Accordingly, bulk isotopic records should primarily reflect signals of the lower photic zone where these organisms dominantly calcify [Stoll, 2005; Stap et al., 2009]. Before the MECO, the vertical water column temperature gradient based on bulk, mixed layer dwelling Acarinina, the subsurface dweller Globigerinatheka, and benthic (~2000 m) δ18O values apparently was ~2–3°C (Figure 4), even less than the gradient calculated for Eocene Thermal Maximum 2 (ETM2) at Site 1263 (~4°C [Stap et al., 2010]). This gradient is much lower than today (~15°C [Levitus et al., 1994]) and lower than in the warm Eocene oceans [Huber and Thomas, 2008]. Deepwater temperature estimates from benthic foraminifera are similar to bulk values from high-latitude Site 689 in the Weddell Sea [Bohaty et al., 2009], which reflect the temperature of upper ocean waters in the region of deepwater formation [e.g., Huber and Thomas, 2008]. At ~41 Ma, just prior to the MECO, high latitudes cooled [Tripati et al., 2005], whereas low latitudes probably remained warm, with surface water temperatures around 30–35°C [Pearson et al., 2007]. Thus, we conclude that our planktic foraminiferal δ18O data cannot be used to approximate mixed layer temperatures [Pearson et al., 2001]. The Acarinina-derived temperatures (mixed layer) appear to be most compromised, lacking a negative δ18O excursion during the MECO. This is not surprising, because Acarinina tests are more porous and thus more readily recrystallized than those of Globigerinatheka [Pearson et al., 2001; Sexton et al., 2006] (see also Figure S2 in the supporting information). In addition, Acarinina were less abundant during MECO [Luciani, unpublished data, 2014], so the lack of a δ18O excursion in analyses of multiple specimens might in part be caused by bioturbational mixing of preevent and postevent specimens, as commonly observed during the Palaeocene–Eocene Thermal Maximum (PETM) [Kelly et al., 1996]. The lack of a δ18O excursion might possibly also be explained by the migration of Acarinina during MECO to greater depths, without losing its symbionts, thus keeping its carbon isotopic composition unchanged. Remaining at constant depth but losing symbionts [e.g., Edgar et al., 2013] could explain the absence of a positive excursion in δ13C during MECO (similar to that in Globigerinatheka) but not the lack of a negative oxygen isotope excursion. We thus conclude that the lack of oxygen isotope excursion in Acarinina most likely was due to recrystallization, as commonly observed [Pearson et al., 2001], possibly combined with bioturbation. Globigerinatheka δ18O values are likely influenced by late ontogenetic (possibly gametogenic) calcite crusts [Sexton et al., 2006], but the relatively positive δ18O values in our records cannot be completely explained by depth habitat [Hollis et al., 2012] also suggesting diagenetic alteration. BOSCOLO GALAZZO ET AL. ©2014. American Geophysical Union. All Rights Reserved. 1153 19449186, 2014, 12, Downloaded from https://agupubs.onlinelibrary.wiley.com/doi/10.1002/2014PA002670 by Tunisia Hinari NPL, Wiley Online Library on [08/12/2024]. See the Terms and Conditions (https://onlinelibrary.wiley.com/terms-and-conditions) on Wiley Online Library for rules of use; OA articles are governed by the applicable Creative Commons License Paleoceanography 10.1002/2014PA002670 TEX86 estimates suggest background SSTs of ~30°C (Figure 2), significantly higher (9–6°C) than the present- day mean annual and summer temperature at the same latitude (~21–24°C [Levitus et al., 1994]), but consistent with middle to late Eocene SST records for middle to low latitudes [Pearson et al., 2007; Liu et al., 2009; Zhang et al., 2013]. The TEX86 record indicates surface water warming between 143 and 138 mcd, corresponding to the MECO interval [Bohaty et al., 2009] (Figure 2). This record, however, shows an earlier onset of warming (Figure 2), not expressed in δ18O records [Bohaty et al., 2009] (Figure 2). TEX86 SSTs are high in the MECO interval but show large fluctuations (Figure 2). These fluctuations might be explained by the ecology of marine Thaumarchaeota, specifically variation in the location of maximum production relative to the thermocline and the chemocline [Pearson and Ingalls, 2013]. On the other hand, the fluctuations in the record might be at least partially explained by bioturbation. Calcareous nannofossil data from the same cores do not suggest evidence of reworking/contamination of the fine fraction due to pervasive bioturbation [Fornaciari et al., 2010], but the sediments may have been disturbed heterogeneously by large burrowers, with some samples possibly representing vertical burrows (halos [Zachos et al., 2004]). Bioturbation would affect the TEX86 record differently than the bulk stable isotope records because of the difference in isoprenoidal GDGT concentrations within/without the MECO and the difference in magnitude of the excursion in TEX86 and stable isotopes. There is a similar uncertainty about primary signal and bioturbation in the explanation of the apparent offsets in the timing of warming by TEX86 and δ18O records. Upper water column indicators (Globigerinatheka and TEX86) consistently record the phases of the MECO lower in the sediment than deeper water indicators (bulk and benthic records; Figure 2). An earlier beginning and longer duration of the peak warming phase in the bulk than in the benthic record (Figure 2) were also observed by Bohaty et al. at Sites 738 and 748 (Figure 3), and a slower recovery in the benthic record (Figure 2) is evident at Site 1218 in the equatorial Pacific and at sites in the Southern Ocean (Figure 3) [Lear et al., 2004; Tripati et al., 2005; Bohaty et al., 2009]. These offsets could reflect actual differences in timing of the phases of the MECO (onset, peak warming, and termination), with a progressive delay of the onset from surface to deeper water (Figure 2), as has been suggested, though not generally accepted, for the PETM [Thomas et al., 2002; Sluijs et al., 2007; Thomas, 2003; Dunkley-Jones et al., 2013]. However, they may also represent bias due to differential bioturbation and/or diagenesis. For instance, GDGTs are associated with the fine-grained sediment fraction [Shah et al., 2008] and can be more easily bioturbated downward than larger particles [Thompson et al., 1995], although the difference between the age of fine-grained sediments and foraminifera is usually only ~1 kyr, i.e., much shorter than differences observed here. Whether or not differential bioturbation can account for the appearance of an earlier onset of warming in the TEX86 record (350 kyr earlier or 2.5 m below) remains speculative. This is the first study coupling planktic stable isotope, benthic stable isotope, and organic proxy temperature data sets for the MECO, so no other comparisons are available. The ~4°C warming in surface and deeper waters (Figures 2 and 4) in our records is similar to that at other sites [Bohaty and Zachos, 2003; Bohaty et al., 2009; Bijl et al., 2010]. Uniform warming of the water column suggests that Southern Ocean surface waters, the probable source of Atlantic intermediate and deep waters [Via and Thomas, 2006; Thomas and Via, 2007; Borelli et al., 2014], warmed to a similar degree as the midlatitude surface waters. Polar amplification during the MECO was thus probably negligible, at least for southern high latitudes [Intergovernmental Panel on Climate Change, 2001; Holland and Bitz, 2003]. 5.2. Repercussions of MECO Warming on Marine Food Webs and Transfer of Organic Carbon to the Seafloor Globigerinatheka δ13C values show a progressive increase approaching the peak warming of the MECO that is not apparent in the bulk δ13C record (Figure 4). Such an increase can result from increased symbiont photosynthetic activity [e.g., Spero and Williams, 1988] and/or reflect lower seawater pH [Spero et al., 1997; Birch et al., 2013; Penman et al., 2014], possibly caused or exacerbated by enhanced water column remineralization of organic matter during warming [John et al., 2013; Ma et al., 2014]. Overall, δ13C records at Site 1263 indicate well-developed water column stratification without substantial changes over the studied time period (Figure 4). Benthic foraminifera and coarse fraction accumulation rates (BFAR and CFAR) increase at the beginning of the record, just below the MECO and up to the basal part of the bulk δ18O negative excursion, then rapidly BOSCOLO GALAZZO ET AL. ©2014. American Geophysical Union. All Rights Reserved. 1154 19449186, 2014, 12, Downloaded from https://agupubs.onlinelibrary.wiley.com/doi/10.1002/2014PA002670 by Tunisia Hinari NPL, Wiley Online Library on [08/12/2024]. See the Terms and Conditions (https://onlinelibrary.wiley.com/terms-and-conditions) on Wiley Online Library for rules of use; OA articles are governed by the applicable Creative Commons License Paleoceanography 10.1002/2014PA002670 decrease, with the lowest rates during peak warming (Figure 6). Our records do not extend sufficiently below the MECO to evaluate whether the increase at the beginning of the record is linked to the gradual warming phase of MECO. The decrease in BFAR during the upper MECO suggests that the amount of organic matter arriving at the seafloor at Site 1263 declined. A parallel decrease in CFAR (Figure 6) could indicate a concomitant decrease in planktic foraminiferal productivity or carbonate dissolution. There is no significant decrease in CaCO3% during MECO at Site 1263 [Bohaty et al., 2009] (Figure 6), and foraminiferal preservation does not show significant changes. In addition, the P/B is high across MECO, showing only small fluctuations (1–2%; Figure 6). We therefore conclude that the decrease in CFAR probably was caused by decreased planktic foraminiferal (i.e., heterotroph) productivity rather than dissolution. We do not think it likely that a decrease in primary autotroph production caused the decline in CFAR and BFAR. Surface waters at Site 1263 would have been consistently nutrient poor due to its location within the subtropical gyre (Figure 1) and well- developed stratification (Figure 4) (see also Winguth et al. for the PETM). Further, FFAR remained constant, indicating that there was no significant change in calcareous nannoplankton productivity (i.e., autotroph primary productivity; Figure 6). We also do not think it likely that a marked decrease in oxygen concentration in the mixed layer or deeper waters caused the decline in CFAR and BFAR. Crenarchaeol, the biomarker of ammonia oxidizing Archaea in the phylum Thaumarchaeota, increased across MECO (Figures 2 and 6), suggesting higher nitrification rates in the water column, for which oxygen is required. Planktic foraminiferal assemblages indicate normal oceanic conditions, and benthic foraminiferal assemblage composition indicates a well- oxygenated seafloor [Boscolo Galazzo et al., 2014]. We suggest that the differential temperature dependency of metabolic rates in photosynthesis and respiration could explain the accumulation rate patterns, as well as the increase in crenarchaeol abundance at Site 1263 across MECO. Biochemical reaction rates, metabolic rates, and nearly all other rates of biological activity increase exponentially with temperature [Arrhenius, 1889; Brown et al., 2004]. This relationship holds for all organisms, autotroph and heterotroph and ectothermic and endothermic, over the biologically relevant temperature range from 0° to 40°C [Gillooly et al., 2001; Brown et al., 2004]. Autotrophs, however, show a lesser increase in metabolic rates than heterotrophs for a specific increase in temperature [O’Connor et al., 2009]. Higher surface and deepwater temperatures during MECO would have increased metabolic rates, thus the demand for carbon and other nutrients to maintain basal metabolism, i.e., the energy required to stay alive [see also Olivarez Lyle and Lyle, 2006; John et al., 2013; Ma et al., 2014]. Constant FFAR across MECO may reflect the lesser response of photosynthetic rates to warming, coupled with population growth limitations imposed by nutrient availability [O’Connor et al., 2009]. The decrease in CFAR and BFAR during MECO may reflect the effect of the greater temperature-dependent increase of metabolic rates in heterotrophs [O’Connor et al., 2009]. Such differential metabolic scaling across trophic levels may have caused the decrease of heterotrophs including foraminifera, as primary productivity during MECO did not match their temperature-enhanced metabolic rates. As an effect of temperature and consumer starvation, the remineralization of organic matter in the water column may have been higher, as has been argued for the PETM [Ma et al., 2014], increasing the concentration of ammonia available for oxidation. This may explain the increase of crenarchaeol across the MECO (Figures 2 and 6), as higher concentration of ammonia may have stimulated nitrification, which in open-ocean areas is mostly mediated by Archea in the phylum Thaumarchaeota [Pearson and Ingalls, 2013]. Increased recycling of organic matter in the water column would have at least reduced the amount of carbon available for sedimentation, further starving benthic foraminifera, as reflected in the decline in BFAR (Figure 6). At Site 1263, as at other sites [Luciani et al., 2010; Edgar et al., 2013], the symbiont-bearing acarininids decreased in abundance across the MECO [Luciani, unpublished data, 2014]. This decrease has been previously attributed to loss of symbionts (bleaching) [Edgar et al., 2013]. Causes of bleaching are poorly known, and potential stress factors include high temperatures, high ultraviolet radiation, hypersalinity, starvation, and acidification [e.g., Douglas, 2003, and references therein]. We argue that starvation due to differential change in metabolic rates of the hosts (Acarinina) and their photosynthetic symbionts could have contributed to the decline of these muricate planktic foraminifera during MECO. BOSCOLO GALAZZO ET AL. ©2014. American Geophysical Union. All Rights Reserved. 1155 19449186, 2014, 12, Downloaded from https://agupubs.onlinelibrary.wiley.com/doi/10.1002/2014PA002670 by Tunisia Hinari NPL, Wiley Online Library on [08/12/2024]. See the Terms and Conditions (https://onlinelibrary.wiley.com/terms-and-conditions) on Wiley Online Library for rules of use; OA articles are governed by the applicable Creative Commons License Paleoceanography 10.1002/2014PA002670 The MECO progressive warming may have thus directly affected the pelagic ecosystem structure at this subtropical gyre location, thus altering pelagic food webs and the recycling of organic carbon. This would have in turn affected the transfer of carbon to the ocean interior through the biological pump, with repercussions for the benthic fauna and the global carbon cycle. Foraminiferal productivity only partially recovered during the cooling after the MECO (Figure 6), which could indicate that a longer time (>800 kyr) was necessary for recovery or that conditions did not return to those before MECO, resulting in overall lower productivity. 5.3. Carbon Isotope Records: Interpreting Global Carbon Cycling Over MECO The carbon isotope signal of calcite tests is less affected by diagenesis and burial than the oxygen isotope signal [e.g., Schrag et al., 1995; Pearson et al., 2001; Sexton et al., 2006]. Although the oxygen isotope signal in Acarinina may be severely compromised, in most samples, its tests have higher δ13C values than deeper calcifying Globigerinatheka (Figure 4), consistent with preferential uptake of 12C during photosynthesis coupled with the biological pump [e.g., Ridgwell, 2011; Sexton et al., 2006]. Bulk δ13C values reflect thermocline depths, with benthic values ~1.5‰ lower (Figures 4 and 5). The primary features of the MECO carbon isotope records [Bohaty et al., 2009] include (1) a progressive, long- term increase in benthic and bulk δ13C paralleling gradual warming; (2) a brief negative CIE coincident with the MECO peak; and (3) an interval of peak positive values in both benthic and bulk records between 39.9 and 39.2 Ma, shortly after the warmer phase. Unlike the PETM and other transient warming events, a significant negative CIE is not associated with the onset of warming [Bohaty and Zachos, 2003; Bohaty et al., 2009; Sluijs et al., 2013]. At Site 1263, benthic δ13C values began to increase at ~40.7 Ma, peaking between 39.9 to 39.2 Ma (Figures 4 and 5), consistent with Southern Ocean benthic records (Figure 5) [Bohaty et al., 2009]. However, our benthic and planktic δ13C records lack a negative CIE coincident with the MECO peak (0.2‰) (Figures 4 and 5). Our bulk δ13C values also lack a pronounced negative CIE (