Guyton Physiology - Pocket Companion - 2015 PDF
Document Details
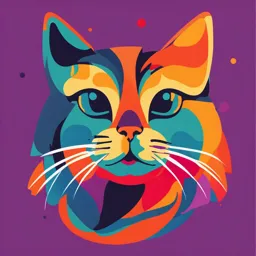
Uploaded by yvesss
Ayura 2027
2015
John E. Hall
Tags
Summary
This book is a pocket-sized companion to Guyton and Hall's Textbook of Medical Physiology (2015). It explains the functions of the human body, from molecules and subcellular components, to organs and systems. The book also examines important topics such as homeostasis and control mechanisms involved in the human body.
Full Transcript
CHAPTER 1 Functional Organization of the Human Body and Control of the “Internal Environment” Physiology is the science that seeks to understand the function of living organisms and their parts. In human phys...
CHAPTER 1 Functional Organization of the Human Body and Control of the “Internal Environment” Physiology is the science that seeks to understand the function of living organisms and their parts. In human physiology, we are concerned with the characteristics of the human body that allow us to sense our environment, move about, think and communicate, reproduce, and perform all of the functions that enable us to survive and thrive as living beings. Human physiology is a broad subject that attempts to explain the specific characteristics and mecha- nisms of the human body that make it a living being. The subject includes the functions of molecules and subcellular components; tissues; organs; organ sys- tems, such as the cardiovascular system; and the interaction and communication among these compo- nents. A distinguishing feature of physiology is that it seeks to integrate the functions of all of the parts of the body to understand the function of the entire human body. Life in the human being relies on this total function, which is considerably more complex than the sum of the functions of the individual cells, tissues, and organs. ois an Cells Are the Living Units of the Body. Each organ aggregate of many cells held together by intercellular supporting structures. The entire body contains about 100 trillion cells, each of which is adapted to perform special functions. These individual cell functions are coordinated by multiple regulatory systems operating in cells, tissues, organs, and organ systems. Although the many cells of the body differ from each other in their special functions, all of them have certain basic characteristics. For example, (1) oxygen combines with breakdown products of fat, carbohy- drates, or protein to release energy that is required for function of the cells; (2) most cells have the abil- ity to reproduce, and whenever cells are destroyed, the remaining cells often regenerate new cells until the appropriate number is restored; and (3) cells are bathed in extracellular fluid, the constituents of which are precisely controlled. 3 4 UNIT I Introduction to Physiology: The Cell and General Physiology MECHANISMS OF HOMEOSTASIS— MAINTENANCE OF NEARLY CONSTANT INTERNAL ENVIRONMENT (p. 4) Essentially all the organs and tissues of the body per- form functions that help maintain the constituents of the extracellular fluid so they are relatively stable, a condition called homeostasis. Much of our discussion of physiology focuses on mechanisms by which the cells, tissues, and organs contribute to homeostasis. Extracellular Fluid Transport and Mixing System—The Blood Circulatory System Extracellular fluid is transported throughout the body in two stages. The first stage is movement of blood throughout the circulatory system, and the second stage is movement of fluid between the blood capil- laries and cells. The circulatory system keeps the flu- ids of the internal environment continuously mixed by pumping blood through the vascular system. As blood passes through the capillaries, a large portion of its fluid diffuses back and forth into the intersti- tial fluid that lies between the cells, allowing continu- ous exchange of substances between the cells and the interstitial fluid and between the interstitial fluid and the blood. Origin of Nutrients in the Extracellular Fluid The respiratory system provides oxygen for the body and removes carbon dioxide. The gastrointestinal system digests food and facili- tates absorption of various nutrients, including car- bohydrates, fatty acids, and amino acids, into the extracellular fluid. The liver changes the chemical composition of many of the absorbed substances to more us- able forms, and other tissues of the body (e.g., fat cells, kidneys, endocrine glands) help modify the absorbed substances or store them until they are needed. The musculoskeletal system consists of skeletal mus- cles, bones, tendons, joints, cartilage, and ligaments. Without this system, the body could not move to the appropriate place to obtain the foods required for nutrition. This system also protects internal organs and supports the body. Functional Organization of the Human Body and Control of the 5 “Internal Environment” Removal of Metabolic End Products (p. 5) The respiratory system not only provides oxygen to the extracellular fluid but also removes carbon diox- ide, which is produced by the cells, released from the blood into the alveoli, and then released to the exter- nal environment. The kidneys excrete most of the waste products other than carbon dioxide. The kidneys play a ma- jor role in regulating extracellular fluid composition by controlling excretion of salts, water, and waste products of the chemical reactions of the cells. By controlling body fluid volumes and compositions, the kidneys also regulate blood volume and blood pressure. The liver eliminates certain waste products pro- duced in the body, as well as toxic substances that are ingested. Regulation of Body Functions The nervous system directs the activity of the muscular system, thereby providing locomotion. It also controls the function of many internal or- gans through the autonomic nervous system, and it allows us to sense our external and internal en- vironment and to be intelligent beings so we can obtain the most advantageous conditions for sur- vival. The hormone systems control many metabolic func- tions of the cells, such as growth, rate of metabolism, and special activities associated with reproduction. Hormones are secreted into the bloodstream and are carried to tissues throughout the body to help regu- late cell function. Protection of the Body The immune system provides the body with a defense mechanism that protects against foreign invaders, such as bacteria and viruses, to which the body is exposed daily. The integumentary system, which is composed mainly of skin, provides protection against injury and defense against foreign invaders, as well as protection of underlying tissues against dehydra- tion. The skin also serves to regulate body temper- ature. 6 UNIT I Introduction to Physiology: The Cell and General Physiology Reproduction The reproductive system provides for formation of new beings like ourselves. Even this function can be consid- ered a homeostatic function because it generates new bodies in which trillions of additional cells can exist in a well-regulated internal environment. CONTROL SYSTEMS OF THE BODY (p. 6) The human body has thousands of control systems that are essential for homeostasis. For example, genetic systems operate in all cells to control intracellular and extracellular functions. Other control systems operate within the organs or throughout the entire body to con- trol interactions among the organs. Regulation of oxygen and carbon dioxide concentra- tions in the extracellular fluid is a good example of multi- ple control systems that operate together. In this instance, the respiratory system operates in association with the nervous system. When carbon dioxide concentration in the blood increases above normal, the respiratory center is excited, causing the person to breathe rapidly and deeply. This breathing increases the expiration of carbon dioxide and therefore removes it from the blood and the extracel- lular fluid until the concentration returns to normal. Normal Ranges of Important Extracellular Fluid Constituents Table 1–1 shows some important constituents of extra- cellular fluid along with their normal values, normal ranges, and maximum limits that can be endured for short periods without the occurrence of death. Note the narrowness of the ranges; levels outside these ranges are usually the cause or the result of illnesses. Characteristics of Control Systems Most Control Systems of the Body Operate by Negative Feedback. For regulation of carbon dioxide concentration, as discussed, a high concentration of carbon dioxide in the extracellular fluid increases pulmonary ventilation, which decreases carbon dioxide concentration, moving it toward normal levels. This mechanism is an example of negative feedback; that is, any stimulus that attempts to change the carbon dioxide concentration is counteracted by a response that is negative to the initiating stimulus. Functional Organization of the Human Body and Control of the 7 “Internal Environment” Table 1–1 Some Important Constituents and Physical Characteristics of the Extracellular Fluid, Normal Range of Control, and Approximate Nonlethal Limits for Short Periods Average Approximate Normal Normal Nonlethal Parameter Units Values Ranges Limits Oxygen (venous) mm Hg 40 35–45 10–1000 Carbon dioxide mm Hg 45 40–50 5–80 (venous) Sodium ion mmol/L 142 138–146 115–175 Potassium ion mmol/L 4.2 3.8–5.0 1.5–9.0 Calcium ion mmol/L 1.2 1.0–1.4 0.5–2.0 Chloride ion mmol/L 106 103–112 70–130 Bicarbonate ion mmol/L 24 22–29 8–45 Glucose mg/dL 90 75–95 20–1500 Body temperature °F (°C) 98.4 98–98.8 65–110 (37.0) (37.0) (18.3–43.3) Acid-base pH 7.4 7.3–7.5 6.9–8.0 The degree of effectiveness with which a control system maintains constant conditions is determined by the gain of the negative feedback. The gain is calculated according to the following formula: Gain = Correction/Error Some control systems, such as those that regulate body temperature, have feedback gains as high as −33, which simply means that the degree of correction is 33 times greater than the remaining error. Feed-Forward Control Systems Anticipate Changes. Because of the many interconnections between control systems, the total control of a particular body function may be more complex than can be accounted for by simple negative feedback. For example, some movements of the body occur so rapidly that there is not sufficient time for nerve signals to travel from some of the peripheral body parts to the brain and then back to the periphery in time to control the movements. Therefore, the brain uses feed-forward control to cause the required muscle contractions. Sensory nerve signals from the moving parts inform the brain in retrospect of whether the appropriate movement, as envisaged by 8 UNIT I Introduction to Physiology: The Cell and General Physiology the brain, has been performed correctly. If it has not, the brain corrects the feed-forward signals it sends to the muscles the next time the movement is required. This process is also called adaptive control, which is, in a sense, delayed negative feedback. Positive Feedback Can Sometimes Cause Vicious Cycles and Death, and Other Times It Can Be Useful. A system that exhibits positive feedback responds to a perturbation with changes that amplify the perturbation and therefore leads to instability rather than stability. For example, severe hemorrhage may lower blood pressure to such a low level that blood flow to the heart is insufficient to maintain normal cardiac pumping; as a result, blood pressure falls even lower, further diminishing blood flow to the heart and causing still more weakness of the heart. Each cycle of this feedback leads to more of the same, which is a positive feedback or a vicious cycle. In some cases the body uses positive feedback to its advantage. An example is the generation of nerve sig- nals. When the nerve fiber membrane is stimulated, the slight leakage of sodium ions into the cell causes open- ing of more channels, more sodium entry, more change in membrane potential, and so forth. Therefore, a slight leak of sodium into the cell becomes an explosion of sodium entering the interior of the nerve fiber, which creates the nerve action potential. SUMMARY—AUTOMATICITY OF THE BODY (p. 10) The body is a social order of about 100 trillion cells orga- nized into various functional structures, the largest of which are called organs. Each functional structure, or organ, helps maintain a constant internal environ- ment. As long as homeostasis is maintained, the cells of the body continue to live and function properly. Thus, each cell benefits from homeostasis and, in turn, each cell contributes its share toward maintenance of homeostasis. This reciprocal interplay provides con- tinuous automaticity of the body until one or more functional systems lose their ability to contribute their share of function. When this loss happens, all the cells of the body suffer. Extreme dysfunction leads to death, whereas moderate dysfunction leads to sickness. CHAPTER 2 The Cell and Its Functions ORGANIZATION OF THE CELL (p. 11) Figure 2–1 shows a typical cell, including the nucleus and cytoplasm, which are separated by the nuclear membrane. The cytoplasm is separated from interstitial fluid by a cell membrane that surrounds the cell. The substances that make up the cell are collectively called protoplasm, which is composed mainly of the following: Water constitutes 70 percent to 85 percent of most cells. Ions/electrolytes provide inorganic chemicals for cel- lular reactions. Some of the most important ions in the cell are potassium, magnesium, phosphate, sul- fate, bicarbonate, and small quantities of sodium, chloride, and calcium. Proteins normally constitute 10 to 20 percent of the cell mass. They can be divided into two types: struc- tural proteins and globular (functional) proteins, which are mainly enzymes. Lipids constitute about 2 percent of the total cell mass. Among the most important lipids in the cells are phospholipids, cholesterol, triglycerides, and neu- tral fats. In adipocytes (fat cells), triglycerides ac- count for as much as 95 percent of the cell mass and represent the body’s main energy storehouse. Carbohydrates play a major role in nutrition of the cell. Most human cells do not store large amounts of carbohydrates, which usually average about 1 percent of the total cell mass but may be as high as 3 percent in muscle cells and 6 percent in liver cells. The small amount of carbohydrates in the cells is usually stored in the form of glycogen, an insoluble polymer of glucose. PHYSICAL STRUCTURE OF THE CELL (p. 12) The cell (Figure 2–1) is not merely a bag of fluid and chemicals; it also contains highly organized physi- cal structures called organelles. Some of the principal organelles of the cell are the cell membrane, nuclear membrane, endoplasmic reticulum (ER), Golgi appara- tus, mitochondria, lysosomes, and centrioles. The Cell and Its Organelles Are Surrounded by Membranes Composed of Lipids and Proteins. The membranes that surround the cell and its organelles 9 10 UNIT I Introduction to Physiology: The Cell and General Physiology Chromosomes and DNA Centrioles Secretory granule Golgi apparatus Microtubules Nuclear Cell membrane membrane Cytoplasm Nucleolus Glycogen Ribosomes Lysosome Mitochondrion Granular Smooth Microfilaments endoplasmic (agranular) reticulum endoplasmic reticulum Figure 2–1 Reconstruction of a typical cell, showing the internal or- ganelles in the cytoplasm and nucleus. include the cell membrane, nuclear membrane, and membranes of the ER, mitochondria, lysosomes, and Golgi apparatus. They provide barriers that prevent free movement of water and water-soluble substances from one cell compartment to another. Proteins in the membrane often penetrate the membrane, providing pathways (channels) to allow movement of specific substances through the membranes. The Cell Membrane Is a Lipid Bilayer With Inserted Proteins. The lipid bilayer is composed almost entirely of phospholipids, sphingolipids, and cholesterol. Phos- pholipids are the most abundant of the cell lipids and have a water-soluble (hydrophilic) portion and a portion that is soluble only in fats (hydrophobic). The hydrophobic portions of the phospholipids face each other, whereas the hydrophilic parts face the two surfaces of the membrane in contact with the surrounding interstitial fluid and the cell cytoplasm. This lipid bilayer membrane is highly permeable to lipid-soluble substances, such as oxygen, carbon diox- ide, and alcohol, but it acts as a major barrier to water- soluble substances, such as ions and glucose. Floating in the lipid bilayer are proteins, most of which are glyco- proteins (proteins combined with carbohydrates). The Cell and Its Functions 11 There are two types of membrane protein: the inte- gral proteins, which protrude through the membrane, and the peripheral proteins, which are attached to the inner surface of the membrane and do not penetrate. - Many of the integral proteins provide structural chan- nels (pores) through which water-soluble substances, especially ions, can diffuse. Other integral proteins act as carrier proteins for the transport of substances, -> sometimes against their gradients for diffusion. -> Integral proteins can also serve as receptors for sub- stances, such as peptide hormones, that do not easily penetrate the cell membrane. The peripheral proteins are normally attached to one > of the integral proteins and usually function as enzymes that catalyze chemical reactions of the cell. The membrane carbohydrates occur mainly in com- bination with proteins and lipids in the form of glyco- proteins and glycolipids. The “glyco” portions of these molecules usually protrude to the outside of the cell. Many other carbohydrate compounds, called proteogly- cans, which are mainly carbohydrate substances bound 2 together by small protein cores, are loosely attached to the outer surface; thus, the entire outer surface of the cell often has a loose carbohydrate coat called the glycocalyx. The carbohydrates on the outer surface of the cell have multiple functions: (1) they are often negatively charged and therefore repel other molecules that are negatively charged; (2) the glycocalyx of cells may attach to other cells (thus the cells attach to each other); (3) some of the carbohydrates act as receptors for binding hormones; and (4) some carbohydrate moieties enter into immune reactions, as discussed in Chapter 35. The Endoplasmic Reticulum Synthesizes Multiple Substances in the Cell. A large network of tubules and vesicles, called the endoplasmic reticulum (ER), penetrates almost all parts of the cytoplasm. The ER membrane provides an extensive surface area for the manufacture of many substances used inside the cells + and released from some cells. They include proteins, carbohydrates, lipids, and other structures such as lysosomes, peroxisomes, and secretory granules. Lipids are made within the ER wall. For the synthesis of proteins, ribosomes attach to the outer surface of the granular ER. These ribosomes function in association with messenger RNA to synthesize many proteins that then enter the Golgi apparatus, where the molecules are further modified before they are released or used in the cell. Part of the ER has ② no attached ribosomes and is called the agranular, or smooth, ER. The agranular ER 12 UNIT I Introduction to Physiology: The Cell and General Physiology functions for the synthesis of lipid substances and for other processes of the cells promoted by intrareticular enzymes. The Golgi Apparatus Functions in Association With the ER. The Golgi apparatus has membranes similar to those of the agranular ER, is prominent in secretory cells, and is located on the side of the cell from which the secretory substances are extruded. Small transport vesicles, also called ER vesicles, continually pinch off from theo ER and-> then fuse with the Golgi apparatus. In this way, substances entrapped in the ER vesicles are transported from the ER to the Golgi apparatus. The substances are then-i processed=in the Golgi apparatus to form lysosomes, secretory vesicles, and other cytoplasmic components. Lysosomes Provide an Intracellular Digestive System. Lysosomes, which are found in great numbers in many cells, are small spherical vesicles surrounded by a membrane that contains digestive enzymes. These enzymes allow lysosomes to break down intracellular substances in structures, especially damaged cell structures, food particles that have been ingested by the cell, and unwanted materials such as bacteria. The membranes surrounding the lysosomes usually prevent the enclosed enzymes from coming in contact with other substances in the cell and therefore prevent their digestive action. When these membranes are dam- aged, the enzymes are released and split the organic substances with which they come in contact into highly diffusible substances such as amino acids and glucose. Mitochondria Release Energy in the Cell. An adequate supply of energy must be available to fuel the chemical reactions of the cell. This energy is provided mainly by the chemical reaction of oxygen with the three types of foods: glucose derived from carbohydrates, fatty acid derived from fats, and amino acids derived from proteins. After entering the cell, foods are split into smaller molecules that, in turn, enter the mitochondria, where other enzymes remove carbon dioxide and hydrogen ions in a process called the citric acid cycle. An oxidative enzyme system, which is also in the mitochondria, causes progressive oxidation of hydrogen atoms. The end products of mitochondria reactions are water and carbon dioxide. The energy liberated is used by mitochondria to synthesize another substance, adenosine triphosphate (ATP), a highly reactive chemical that can diffuse throughout the cell to release its energy whenever it is needed for the performance of cell functions. The Cell and Its Functions 13 Mitochondria are also self-replicative, which means that one mitochondrion can form a second one, a third one, and so on whenever there is a need in the cell for increased amounts of ATP. There Are Many Cytoplasmic Structures and Organelles. Hundreds of types of cells are found in the body, and each has a special structure. Some cells, for example, are rigid and have large numbers of filamentous or tubular structures, which are composed of fibrillar proteins. A major function of these tubular structures is to act as a cytoskeleton, providing rigid physical structures for certain parts of cells. Some of the tubular structures, called microtubules, can transport substances from one area of the cell to another. One of the important functions of many cells is to secrete special substances, such as digestive enzymes. Almost all of the substances are formed by the ER- Golgi apparatus system and are released into the cyto- plasm inside storage vesicles called secretory vesicles. After a period of storage in the cell, they are expelled through the cell membrane to be used elsewhere in the body. The Nucleus Is the Control Center of the Cell and Contains Large Amounts of DNA, Also Called Genes (p. 17). Genes determine the characteristics of the proteins of the cell, including the enzymes of the cytoplasm. They also control reproduction. Genes first reproduce themselves through a process of mitosis in which two daughter cells are formed, each of which receives one of the two sets of genes. The nuclear membrane, also called the nuclear enve- lope, separates the nucleus from the cytoplasm. This structure is composed of two membranes; the outer membrane is continuous with the ER, and the space between the two nuclear membranes is also continuous with the compartment inside the ER. Both layers of the membrane are penetrated by several thousand nuclear pores, which are almost 100 nanometers in diameter. The nuclei in most cells contain one or more structures called nucleoli, which, unlike many of the organelles, do not have a surrounding membrane. The nucleoli contain large amounts of RNA and proteins of the type found in ribosomes. A nucleolus becomes enlarged when the cell is actively synthesizing proteins. Ribosomal RNA is stored in the nucleolus and trans- ported through the nuclear membrane pores to the cytoplasm, where it is used to produce mature ribo- somes, which play an important role in the formation of proteins. 14 UNIT I Introduction to Physiology: The Cell and General Physiology FUNCTIONAL SYSTEMS OF THE CELL (p. 19) Ingestion by the Cell—Endocytosis The cell obtains nutrients and other substances from the surrounding fluid through the cell membrane via diffusion and active transport. Very large particles · enter the cell viaoendocytosis, the principal forms of which are pinocytosis and phagocytosis. Pinocytosis is the ingestion of small globules of ex- tracellular fluid, forming minute vesicles in the cell cytoplasm. This process is the only method by which large molecules, such as proteins, can enter the cells. These molecules usually attach to specialized recep- tors on the outer surface of the membrane that are concentrated in small pits called coated pits. On the inside of the cell membrane underneath these pits is a latticework of a fibrillar protein called clathrin and a contractile filament of actin and myosin. After the protein molecules bind with the receptors, the mem- brane invaginates and contractile proteins surround the pit, causing its borders to close over the attached proteins and form a pinocytotic vesicle. Phagocytosis is the ingestion of large particles, such as bacteria, cells, and portions of degenerating tissue. This ingestion occurs much in the same way as pino- cytosis except that it involves large particles instead of molecules. Only certain cells have the ability to perform phagocytosis, notably tissue macrophages and some white blood cells. Phagocytosis is initiated when proteins or large polysaccharides on the sur- face of the particle bind with receptors on the sur- face of the phagocyte. In the case of bacteria, these usually are attached to specific antibodies, and the antibodies in turn attach to the phagocyte receptors, dragging the bacteria along with them. This inter- mediation of antibodies is called opsonization and is discussed further in Chapters 34 and 35. Pinocytic and Phagocytic Foreign Substances Are Digested in the Cell by the Lysosomes. Almost as soon as pinocytic or phagocytic vesicles appear inside a cell, lysosomes become attached to the vesicles and empty their digestive enzymes into the vesicle. Thus, a digestive vesicle is formed in which the enzymes begin hydrolyzing the proteins, carbohydrates, lipids, and other substances in the vesicle. The products of digestion are small molecules of amino acids, glucose, phosphate, and so on that can diffuse through the membrane of the vesicle into the cytoplasm. The undigested substances, called the residual body, are excreted through the The Cell and Its Functions 15 cell membrane via the process of exocytosis, which is basically the opposite of endocytosis. Synthesis of Cellular Structures by ER and Golgi Apparatus (p. 20) The Synthesis of Most Cell Structures Begins in the ER. Many of the products formed in the ER are then passed onto the Golgi apparatus, where they are further processed before release into the cytoplasm. The granular ER, characterized by large numbers of ⑥ ribosomes attached to the outer surface, is the site of protein formation. Ribosomes synthesize the proteins and extrude many of them through the wall of the ER to the interior of the endoplasmic vesicles and tubules, called the endoplasmic matrix. When proteins enter the ER, enzymes in the ER wall cause rapid changes, including congregation of carbo- hydrates to form glycoproteins. In addition, the proteins are often cross-linked, folded, and shortened to form more compact molecules. The ER also synthesizes lipids, especially phospho- lipid and cholesterol, which are incorporated into the lipid bilayer of the ER. Small ER vesicles, or transport vesicles, continually break off from the smooth reticu- lum. Most of these vesicles migrate rapidly to the Golgi apparatus. The Golgi Apparatus Processes Substances Formed in the ER. As substances are formed in the ER, especially proteins, they are transported through the reticulum tubules toward the portions of the smooth ER that lie nearest the Golgi apparatus. Small transport vesicles, composed of small envelopes of smooth ER, continually break away and diffuse to the deepest layer of the Golgi apparatus. The transport vesicles instantly fuse with the Golgi apparatus and empty their contents into the vesicular spaces of the Golgi apparatus. Here, more carbohydrates are added to the secretions, and the ER secretions are compacted. As the secretions pass toward the outermost layers of the Golgi apparatus, the compaction and processing continue. Finally, small and large vesicles break away from the Golgi apparatus, carrying with them the compacted secretory substances. These substances can then diffuse throughout the cell. In a highly secretory cell, the vesicles formed by the Golgi apparatus are mainly secretory vesicles, which dif- fuse to the cell membrane, fuse with it, and eventually empty their substances to the exterior via a mechanism called exocytosis. Some of the vesicles made in the Golgi 16 UNIT I Introduction to Physiology: The Cell and General Physiology apparatus, however, are destined for intracellular use. For example, specialized portions of the Golgi appara- tus form lysosomes. Extraction of Energy From Nutrients by the Mitochondria (p. 22) The principal substances from which the cells extract energy are oxygen and one or more of the foodstuffs— carbohydrates, fats, and proteins—that react with oxy- gen. In humans, almost all carbohydrates are converted to glucose by the digestive tract and liver before they reach the cell; similarly, proteins are converted to amino acids, and fats are converted to fatty acids. Inside the cell, these substances react chemically with oxygen under the influence of enzymes that control the rates of reaction and channel the released energy in the proper direction. Oxidative Reactions Occur Inside the Mitochondria, and Energy Released Is Used to Form ATP. ATP is a nucleotide composed of the nitrogenous base adenine, the pentose sugar ribose, and three phosphate radicals. The last two phosphate radicals are connected with the remainder of the molecule by high-energy phosphate bonds, each of which contains about 12,000 calories of energy per mole of ATP under the usual conditions of the body. The high-energy phosphate bonds are labile so they can be split instantly whenever energy is required to promote other cellular reactions. When ATP releases its energy, a phosphoric acid radical is split away, and adenosine diphosphate (ADP) is formed. Energy derived from cell nutrients causes the ADP and phosphoric acid to recombine to form new ATP, with the entire process continuing over and over again. Most of the ATP Produced in the Cell Is Formed in Mitochondria. After entry into the cells, glucose is < subjected to enzymes in the cytoplasm that convert it to pyruvic acid, a process called glycolysis. Less than 5 percent of the ATP formed in the cell occurs via glycolysis. Pyruvic acid derived from carbohydrates, fatty acids derived from lipids, and amino acids derived from proteins ↓are all eventually converted to the compound acetyl–coenzyme A (acetyl-CoA) in the mitochondria matrix. This substance is then acted on by another series of enzymes in a sequence of chemical reactions called the citric acid cycle, or Krebs cycle. In the citric acid cycle, acetyl-CoA is split into hydro- gen ions and carbon dioxide. Hydrogen ions are highly The Cell and Its Functions 17 reactive and eventually combine with oxygen that has diffused into the mitochondria. This reaction releases a tremendous amount of energy, which is used to convert large amounts of ADP to ATP. This requires large num- bers of protein enzymes that are integral parts of the mitochondria. The oinitial event in ATP formation is removal of an electron from the hydrogen atom, thereby converting it to a hydrogen ion. The o terminal event is movement of the hydrogen ion through large globular proteins called ATP synthetase, which protrude through the mem- branes of the mitochondrial membranous shelves, which themselves protrude into the mitochondrial matrix. ATP synthetase is an enzyme that uses the energy and movement of the hydrogen ions to effect the conver- sion of ADP to ATP, and hydrogen ions combine with oxygen to form water. The newly formed ATP is trans- ported out of the mitochondria to all parts of the cell cytoplasm and nucleoplasm, where it is used to energize the functions of the cell. This overall process is called the chemosmotic mechanism of ATP formation. ATP Is Used for Many Cellular Functions. ATP promotes three types of cell function: (1) membrane transport, as occurs with the sodium-potassium pump, which transports sodium out of the cell and potassium into the cell; (2) synthesis of chemical compounds throughout the cell; and (3) mechanical work, as occurs with the contraction of muscle fibers or with ciliary and ameboid motion. Locomotion and Ciliary Movements of Cells (p. 24) The most obvious type of movement in the body is that of the specialized muscle cells in skeletal, cardiac, and smooth muscle, which constitute almost 50 percent of the entire body mass. Two other types of movement occur in other cells: ameboid locomotion and ciliary movement. Ameboid Movement of an Entire Cell in Relation to Its Surroundings. An example of ameboid locomotion is the movement of white blood cells through tissues. Typically, ameboid locomotion begins with protrusion of a pseudopodium from one end of the cell. This results from continual exocytosis, which forms a new cell membrane at the leading edge of the pseudopodium, and continual endocytosis of the membrane in the mid and rear portions of the cell. Two other effects are also essential to the forward ⑦ movement of the cell. The first effect is attachment 18 UNIT I Introduction to Physiology: The Cell and General Physiology of the pseudopodium to the surrounding tissues so it becomes fixed in its leading position while the remain- der of the cell body is pulled forward toward the point of attachment. This attachment is effected by receptor proteins that line the insides of the exocytotic vesicles. ① The second requirement for locomotion is avail- able energy needed to pull the cell body in the direction of the pseudopodium. In the cytoplasm of all cells are molecules of the protein actin. When these molecules polymerize to form a filamentous network, the net- work contracts when it binds with another protein, for example, an actin-binding protein such as myosin. The entire process, which is energized by ATP, takes place in the pseudopodium of a moving cell, in which such a network of actin filaments forms inside the growing pseudopodium. The most important factor that usually initiates ame- boid movement is the process called chemotaxis, which results from the appearance of certain chemical sub- stances in the tissue called chemotactic substances. Ciliary Movement Is a Whiplike Movement of Cilia on the Surfaces of Cells. Ciliary movement occurs in only two places in the body: on the inside surfaces of the respiratory airways and on the inside surfaces of the uterine tubes (i.e., the fallopian tubes of the reproductive tract). In the nasal cavity and lower respiratory airways, the whiplike motion of the cilia causes a layer of mucus to move toward the pharynx at a rate of about 1 cm/min; in this way, passageways with mucus or particles that become entrapped in the mucus are continually cleared. In the uterine tubes, the cilia cause slow movement of fluid from the ostium of the uterine tube toward the uterine cavity; it is mainly this movement of fluid that transports the ovum from the ovary to the uterus. The mechanism of the ciliary movement is not fully understood, but at least two factors are necessary: (1) available ATP and (2) appropriate ionic conditions, including appropriate concentrations of magnesium and calcium. CHAPTER 3 Genetic Control of Protein Synthesis, Cell Function, and Cell Reproduction Genes in the Cell Nucleus Control Protein Synthesis (p. 27). The genes control protein synthesis in the cell and in this way control cell function. Proteins play a key role in almost all functions of the cell by serving as enzymes that catalyze the reactions of the cell and as major components of the physical structures of the cell. Each gene is a double-stranded, helical molecule of deoxyribonucleic acid (DNA) that controls formation "G of ribonucleic acid (RNA). The RNA, in turn, spreads throughout the cells to control the formation of a spe- cific protein. The entire process, from transcription of the genetic code in the nucleus to translation of the RNA code and formation of proteins in the cell cytoplasm, is often referred to as gene expression and is shown in Figure 3–1. Because there are about 30,000 genes in each cell, it is possible to form large numbers of different cel- lular proteins. In fact, RNA molecules transcribed from Plasma Nuclear membrane envelope Nucleus DNA Gene (DNA) DNA Transcription transcription RNA RNA formation RNA splicing Translation mRNA Ribosomes RNA transport Protein mRNA formation Translation of messenger RNA Cell Cell structure enzymes Protein Cytosol Cell function Figure 3–1 General schema by which the genes control cell function. 19 20 UNIT I Introduction to Physiology: The Cell and General Physiology the same gene can be processed in different ways by the cell, giving rise to alternate versions of the protein. The total number of different proteins produced by various cell types in humans is estimated to be at least 100,000. Nucleotides Are Organized to Form Two Strands of DNA Loosely Bound to Each Other. Genes are attached in an end- on-end manner in long, double-stranded, helical molecules of DNA that are composed of three basic building blocks: (1) phosphoric acid, (2) deoxyribose (a sugar), and (3) four nitrogenous bases: two purines (adenine and guanine) and two pyrimidines (thymine and cytosine). The first stage in DNA formation is the combina- tion of one molecule of phosphoric acid, one molecule of deoxyribose, and one of four bases to form a nucleo- tide. Four nucleotides can therefore be formed, one from each of the four bases. Multiple nucleotides are bound together to form two strands of DNA, and the two strands are loosely bound to each other. The backbone of each DNA strand is composed of alternating phosphoric acid and deoxyribose molecules. The purine and pyrimidine bases are attached to the side of the deoxyribose molecules, and loose bonds between the purine and pyrimidine bases of the two DNA strands hold them together. The purine base adenine of one strand always bonds with the pyrimidine base thymine of the other strand, whereas guanine always bonds with cytosine. The Genetic Code Consists of Triplets of Bases. Each group of three successive bases in the DNA strand is called a code word. These code words control the sequence of amino acids in the protein to be formed in the cytoplasm. One code word, for example, might be composed of a sequence of adenine, thymine, and guanine, whereas the next code word might have a sequence of cytosine, guanine, and thymine. These two code words have entirely different meanings because their bases are different. The sequence of successive code words of the DNA strand is known as the genetic code. THE DNA CODE IN THE NUCLEUS IS TRANSFERRED TO RNA CODE IN THE CELL CYTOPLASM—THE PROCESS OF TRANSCRIPTION (p. 30) Because DNA is located in the nucleus and many func- tions of the cell are carried out in the cytoplasm, there must be some method by which the genes of the nucleus control the chemical reactions of the cytoplasm. This is achieved through RNA, the formation of which is con- trolled by DNA. During this process the code of DNA is transferred to RNA, a process called transcription. Genetic Control of Protein Synthesis, Cell Function, and Cell 21 Reproduction The RNA diffuses from the nucleus to the nuclear pores into the cytoplasm, where it controls protein synthesis. RNA Is Synthesized in the Nucleus From a DNA Template. During synthesis of RNA, the two strands of the DNA molecule separate, and one of the two strands is used as a template for RNA synthesis. The code triplets in DNA cause the formation of complementary code triplets (called codons) in RNA; these codons then control the sequence of amino acids in a protein to be synthesized later in the cytoplasm. Each DNA strand in each chromosome carries the code for perhaps as many as 2000 to 4000 genes. The basic building blocks of RNA are almost the same as those of DNA except that in RNA, the sugar ribose replaces the sugar deoxyribose and the pyrimi- dine uracil replaces thymine. The basic building blocks of RNA combine to form four nucleotides, exactly as described for the synthesis of DNA. These nucleotides contain the bases adenine, guanine, cytosine, and uracil. The next step in the RNA synthesis is activation of the nucleotides, which occurs through the addition of two phosphate radicals to each nucleotide to form tri- phosphates. These last two phosphates are combined with the nucleotide by high-energy phosphate bonds, which are derived from the adenosine triphosphate (ATP) of the cell. This activation process makes avail- able large quantities of energy, which is used for pro- moting the chemical reactions that add each new RNA nucleotide to the end of the RNA chain. The DNA Strand Is Used as a Template to Assemble the RNA Molecule From Activated Nucleotides. The assembly of the RNA molecule occurs under the influence of the enzyme RNA polymerase as follows: 1. In the DNA strand immediately ahead of the gene that is to be transcribed is a sequence of nucleotides called the promoter. An RNA polymerase recognizes this promoter and attaches to it. 2. The polymerase causes unwinding of two turns of the DNA helix and separation of the unwound portions. 3. The polymerase moves along the DNA strand and begins forming the RNA molecules by binding com- plementary RNA nucleotides to the DNA strand. 4. The successive RNA nucleotides then bind to each other to form an RNA strand. 5. When the RNA polymerase reaches the end of the DNA gene, it encounters a sequence of DNA mole- cules called the chain-terminating sequence, caus- ing the polymerase to break away from the DNA strand. The RNA strand is then released into the nucleoplasm. 22 UNIT I Introduction to Physiology: The Cell and General Physiology The code present in the DNA strand is transmitted in complementary form to the RNA molecule as fol- lows: DNA Base RNA Base Guanine Cytosine Cytosine Guanine Adenine Uracil Thymine Adenine There Are Several Types of RNA. Research on RNA has uncovered many different types of RNA. Some are involved in protein synthesis, whereas others serve gene regulatory functions or are involved in posttranscriptional modification of RNA. The following six types of RNA play independent and different roles in protein synthesis: 1. Precursor messenger RNA (pre-mRNA), a large, im- mature single strand of RNA that is processed in the nucleus to form mature mRNA and includes two different types of segments called introns, which are removed by a process called splicing, and exons, which are retained in the final mRNA 2. Small nuclear RNA (snRNA), which directs the splicing of pre-mRNA to form mRNA 3. mRNA, which carries the genetic code to the cyto- plasm to control the formation of proteins 4. ribosomal RNA, which, along with proteins, forms the ribosomes, the structures in which protein mol- ecules are assembled 5. Transfer RNA (tRNA), which transports activated amino acids to the ribosomes to be used in the as- sembly of the proteins 6. microRNA (miRNA), which are single-stranded RNA molecules of 21 to 23 nucleotides that can regulate gene transcription and translation There are 20 types of tRNA, each of which combines specifically with one of the 20 amino acids and carries this amino acid to the ribosomes, where it is incorporated in the protein molecule. The code in the tRNA that allows it to recognize a specific codon is a triplet of nucleotide bases called an anticodon. During formation of the pro- tein molecule, the three anticodon bases combine loosely by hydrogen bonding with the codon bases of the mRNA. In this way, the various amino acids are lined up along the mRNA chain, thus establishing the proper sequence of amino acids in the protein molecule. Genetic Control of Protein Synthesis, Cell Function, and Cell 23 Reproduction TRANSLATION—SYNTHESIS OF POLYPEPTIDES ON RIBOSOMES FROM GENETIC CODE IN mRNA (p. 33) To manufacture proteins, one end of the mRNA strand enters the ribosome, and then the entire strand threads its way through the ribosome in just over a minute. As it passes through, the ribosome “reads” the genetic code and causes the proper succession of amino acids to bind together to form chemical bonds called peptide linkages. The mRNA does not recognize the different types of amino acids but, instead, recognizes the different types of tRNA. Each type of tRNA molecule carries only one specific type of amino acid that is incorporated into the protein. Thus, as the strand of mRNA passes through the ribo- some, each of its codons attracts to it a specific tRNA that, in turn, delivers a specific amino acid. This amino acid then combines with the preceding amino acids to form a peptide linkage, and this sequence continues to build until an entire protein molecule is formed. At this point, a chain-terminating (or “stop”) codon appears and indicates completion of the process, and the protein is released into the cytoplasm or through the membrane of the endoplasmic reticulum to the interior. CONTROL OF GENE FUNCTION AND BIOCHEMICAL ACTIVITY IN CELLS (p. 35) The genes control the function of each cell by determin- ing the relative proportion of various types of enzymes and structural proteins that are formed. Regulation of gene expression covers the entire process from tran- scription of the genetic code in the nucleus to the for- mation of proteins in the cytoplasm. The Promoter Controls Gene Expression. Cellular protein synthesis starts with transcription of DNA into RNA, a process controlled by regulatory elements in the promoter of a gene. In eukaryotes, including mammals, the basal promoter consists of a sequence of seven bases (TATAAAA) called the TATA box, which is the binding site for the TATA-binding protein and several other important transcription factors that are collectively referred to as the transcription factor IID complex. In addition to the transcription factor IID complex, this region is where transcription factor IIB binds to both the DNA and RNA polymerase 2 to facilitate transcription of the DNA into RNA. This basal promoter is found in all protein coding genes, 24 UNIT I Introduction to Physiology: The Cell and General Physiology and the polymerase must bind with this basal promoter before it can begin traveling along the DNA strand to synthesize RNA. The upstream promoter is located further upstream from the transcription start site and contains several binding sites for positive or negative transcription factors that can effect transcription through interactions with proteins bound to the basal promoter. The structure and transcription factor binding sites in the upstream promoter vary from gene to gene to give rise to the different expression patterns of genes in different tissues. Transcription of genes in eukaryotes is also influ- enced by enhancers, which are regions of DNA that can bind transcription factors. Enhancers can be located far from the gene they act on or even on a different chro- mosome. Although enhancers may be located a great distance away from their target gene, they may be rela- tively close when DNA is coiled in the nucleus. It is esti- mated that there are 110,000 gene enhancer sequences in the human genome. Control of the Promoter Through Negative Feedback by the Cell Product. When the cell produces a critical amount of substance, it causes negative feedback inhibition of the promoter that is responsible for its synthesis. This inhibition can be accomplished by causing a regulatory repressor protein to bind at the repressor operator or a regulatory activator protein to break this bond. In either case, the promoter becomes inhibited. Other mechanisms are available for control of tran- scription by the promoter, including the following: 1. A promoter may be controlled by transcription fac- tors located elsewhere in the genome. 2. In some instances, the same regulatory protein func- tions as an activator for one promoter and as a re- pressor for another, allowing different promoters to be controlled at the same time by the same regula- tory protein. 3. The nuclear DNA is packaged in specific structural units, the chromosomes. Within each chromosome, the DNA is wound around small proteins called his- tones, which are held together tightly in a compacted state with other proteins. As long as DNA is in this compacted state, it cannot function to form RNA. Multiple mechanisms exist, however, that can cause selected areas of the chromosomes to become de- compacted, allowing RNA transcription. Even then, specific transcription factors control the actual rate of transcription by the promoter in the chromo- some. Genetic Control of Protein Synthesis, Cell Function, and Cell 25 Reproduction THE DNA–GENETIC SYSTEM CONTROLS CELL REPRODUCTION (p. 37) The genes and their regulatory mechanisms determine not only the growth characteristics of cells but also when and whether these cells divide to form new cells. In this way, the genetic system controls each stage of the development of the human from the single-cell fertil- ized ovum to the whole functioning body. Most cells of the body, with the exception of mature red blood cells, striated muscle cells, and neurons, are capable of reproducing other cells of their own type. Ordinarily, as sufficient nutrients are available, each cell increases in size until it divides via mitosis to form two new cells. Different cells of the body have different life cycle periods that vary from as short as 10 hours for highly stimulated bone marrow cells to the entire life- time of the human body for nerve cells. Cell Reproduction Begins With Replication of DNA. Mitosis can take place only after all of the DNA in the chromosomes has been replicated. The DNA is duplicated only once, so the net result is two exact replicates of all DNA. These replicates then become the DNA of the two daughter cells that will be formed at mitosis. The replication of DNA is similar to the way RNA is transcribed from DNA, except for a few important differences: 1. Both strands of the DNA are replicated, not just one of them. 2. Both strands of the DNA helix are replicated from end to end rather than small portions of them, as oc- curs during the transcription of RNA by genes. 3. The principal enzymes for replication of DNA are a complex of several enzymes called DNA polymerase, which is comparable to RNA polymerase. 4. Each newly formed DNA strand remains attached by loose hydrogen bonding to the original DNA strand that is used as its template. Two DNA helixes that are formed, therefore, are duplicates of each other and are still coiled together. 5. The two new helixes become uncoiled by the action of enzymes that periodically cut each helix along its entire length, rotate each segment sufficiently to cause separation, and then resplice the helix. DNA Strands Are “Repaired” and “Proofread.” During the time between the replication of DNA and the beginning of mitosis, there is a period of “proofreading” and “repair” of the DNA strands. Whenever inappropriate DNA nucleotides have been matched up with the nucleotides 26 UNIT I Introduction to Physiology: The Cell and General Physiology of the original template strand, special enzymes cut out the defective areas and replace them with the appropriate complementary nucleotides. Because of proofreading and repair, the transcription process rarely makes a mistake. When a mistake is made, however, it is called a mutation. Entire Chromosomes Are Replicated. The DNA helixes of the nucleus are each packaged as a single chromosome. The human cell contains 46 chromosomes arranged in 23 pairs. In addition to the DNA in the chromosome, there is a large amount of protein composed mainly of histones, around which small segments of each DNA helix are coiled. During mitosis, the successive coils are packed against each other, allowing the long DNA molecule to be packaged in a coiled and folded arrangement. Replication of the chromosomes in their entirety occurs soon after replication of the DNA helixes. The two newly formed chromosomes remain temporarily attached to each other at a point called the centromere, which is located near their center. These duplicated but still-attached chromosomes are called chromatids. Mitosis Is the Process by Which the Cell Splits Into Two New Daughter Cells. Two pairs of centrioles, which are small structures that lie close to one pole of the nucleus, begin to move apart from each other. This movement is caused by successive polymerization of protein microtubules growing outward from each pair of centrioles. As the tubules grow, they push one pair of centrioles toward one pole of the cell and the other toward the opposite pole. At the same time, other microtubules grow radially away from each of the centriole pairs, forming a spiny star called the aster at each end of the cell. The complex of microtubules extending between the centriole pairs is called the spindle, and the entire set of microtubules plus the pairs of centrioles is called the mitotic apparatus. Mitosis then proceeds through several phases. Prophase is the beginning of mitosis. While the spindle is forming, the chromosomes of the nucleus become condensed into well-defined chromosomes. Prometaphase is the stage at which the growing mi- crotubular spines of the aster puncture and fragment the nuclear envelope. At the same time, the microtu- bules from the aster become attached to the chroma- tids at the centromere, where the paired chromatids are still bound to each other. Metaphase is the stage at which the two asters of the mitotic apparatus are pushed farther and farther Genetic Control of Protein Synthesis, Cell Function, and Cell 27 Reproduction apart by additional growth of the mitotic spindle. Simultaneously, the chromatids are pulled tightly by the attached microtubules to the center of the cell, lining up to form the equatorial plate of the mitotic spindle. Anaphase is the stage at which the two chromatids of each chromosome are pulled apart at the centro- mere. Thus all 46 pairs of chromosomes are separat- ed, forming two sets of 46 daughter chromosomes. Telophase is the stage at which the two sets of daugh- ter chromosomes are pulled completely apart. Then the mitotic apparatus dissolves, and a new nuclear membrane develops around each set of chromo- somes. Cell Differentiation Allows Different Cells of the Body to Perform Different Functions. As a human develops from a fertilized ovum, the ovum divides repeatedly until trillions of cells are formed. The new cells gradually differentiate from each other, with certain cells having different genetic characteristics from other cells. This differentiation process occurs as a result of inactivation of certain genes and activation of others during successive stages of cell division. This process of differentiation leads to the ability of different cells in the body to perform different functions. This page intentionally left blank UNIT II Membrane Physiology, Nerve, and Muscle 4 Transport of Substances Through Cell Membranes, 31 5 Membrane Potentials and Action Potentials, 38 6 Contraction of Skeletal Muscle, 44 7 Excitation of Skeletal Muscle: Neuromuscular Transmission and Excitation-Contraction Coupling, 51 8 Excitation and Contraction of Smooth Muscle, 55 This page intentionally left blank CHAPTER 4 Transport of Substances Through Cell Membranes Differences between the composition of intracellular and extracellular fluids are caused by transport mecha- nisms of cell membranes. Major differences in composi- tion include the following: Extracellular fluid has higher concentrations of so- dium, calcium, bicarbonate, and chloride, compared with intracellular fluid. Intracellular fluid has higher concentrations of po- tassium, phosphates, magnesium, and proteins com- pared with extracellular fluid. The Cell Membrane Consists of a Lipid Bilayer Containing Many Different Protein Molecules. The lipid bilayer constitutes a barrier for movement of most water-soluble substances. However, smaller, lipid- soluble substances can pass directly through the lipid bilayer. Protein molecules in the lipid bilayer constitute an alternate transport pathway for water- soluble substances. Channel proteins provide a watery pathway for movement of (mainly) ions across the membrane. Carrier proteins bind I with specific molecules and then undergo conformational changes that move molecules across the membrane. Transport Through the Cell Membrane Occurs Via Diffusion or Active Transport. Diffusion means random movement of molecules ei- ther through intermolecular spaces in the cell mem- brane or in combination with a carrier protein. The energy that causes diffusion is the energy of the nor- mal kinetic motion of matter. Active transport means movement of substances across the membrane in combination with a carrier protein and also against an electrochemical gradient. This process requires a source of energy in addition to kinetic energy. DIFFUSION (p. 47) Diffusion Is the Continual Movement of Molecules in Liquids or Gases. Diffusion through the cell membrane can be divided into the following two subtypes: Simple diffusion means that molecules move through a membrane without binding to carrier proteins. Simple diffusion can occur by way of two pathways: 31 32 UNIT II Membrane Physiology, Nerve, and Muscle (1) through the interstices of the lipid bilayer, and (2) through water-filled protein channels that span the cell membrane. Facilitated diffusion requires a carrier protein. The carrier protein aids in passage of molecules through the membrane, probably by binding chemically with them and shuttling them through the membrane in this form. The Rate of Diffusion of a Substance Through the Cell Membrane Is Directly Proportional to Its Lipid Solubility. The lipid solubilities of oxygen, nitrogen, carbon dioxide, anesthetic gases, and most alcohols are so high that they can diffuse directly through the lipid bilayer of the cell membrane. Water and Other Lipid-Insoluble Molecules, Mainly Ions, Diffuse Through Protein Channels in the Cell Membrane. Water readily penetrates the cell membrane and can also pass through transmembrane protein channels. Other lipid-insoluble molecules (mainly ions) of a sufficiently small size can pass through the water-filled protein channels. Protein Channels Have Selective Permeability for Transport of One or More Specific Molecules. The selective permeability of protein channels results from the characteristics of the channel itself, such as its diameter, its shape, and the nature of the electrical charges along its inner surfaces. Gating of Protein Channels Provides a Means for Controlling Their Permeability. The gates are thought to be molecular extensions of the transport protein, which can close over the channel opening or be lifted from the opening by a conformational change in the protein molecule itself. The opening and closing of gates are controlled in two principal ways: Voltage gating. In this instance, the molecular con- formation of the gate is controlled by the electrical potential across the cell membrane. For example, the normal negative charge on the inside of the cell membrane causes sodium gates to remain tightly closed. When the inside of the membrane loses its negative charge (i.e., becomes less negative), these gates open, allowing sodium ions to pass inward through the sodium channels. The opening of sodi- um channel gates initiates action potentials in nerve fibers. Chemical gating. Some protein channel gates are opened by the binding of another molecule with the protein, which causes a conformational change in the membrane protein that opens or closes the Transport of Substances Through Cell Membranes 33 gate. This process is called chemical (or ligand) gating. One of the most important instances of chemical gating is the effect of acetylcholine on the “acetylcholine cation channel” of the neuro- muscular junction. Facilitated Diffusion Is Also Called Carrier-Mediated Diffusion. Molecules transported by facilitated diffusion usually cannot pass through the cell membrane without the assistance of a specific carrier protein. Facilitated diffusion involves the following two steps: (1) the molecule to be transported enters a blind-ended channel and binds to a specific recep- tor, and (2) a conformational change occurs in the carrier protein, so the channel now opens to the opposite side of the membrane where the molecule is deposited. Facilitated diffusion differs from simple diffusion in the following important way. The rate of simple diffusion increases proportionately with the concen- tration of the diffusing substance. With facilitated diffusion, the rate of diffusion approaches a maxi- mum value as the concentration of the substance in- creases. This maximum rate is dictated by the rate at which the carrier protein molecule can undergo the conformational change. Among the most important substances that cross cell membranes by facilitated diffusion are glucose and most of the amino acids. Factors That Affect Net Rate of Diffusion (p. 52) Substances Can Diffuse in Both Directions Through the Cell Membrane. Therefore, what is usually important is the net rate of diffusion of a substance in one direction. This net rate is determined by the following factors: Permeability. The permeability of a membrane for a given substance is expressed as the net rate of diffu- sion of the substance through each unit area of the membrane for a unit concentration difference be- tween the two sides of the membrane (when there are no electrical or pressure differences). Concentration difference. The rate of net diffusion through a cell membrane is proportional to the dif- ference in concentration of the diffusing substance on the two sides of the membrane. Electrical potential. If an electrical potential is ap- plied across a membrane, the ions move through the membrane because of their electrical charges. When large amounts of ions have moved through the 34 UNIT II Membrane Physiology, Nerve, and Muscle membrane, a concentration difference of the same ions develops in the direction opposite to the elec- trical potential difference. When the concentration difference rises to a sufficiently high level, the two effects balance each other, creating a state of electro- chemical equilibrium. The electrical difference that balances a given concentration difference can be cal- culated using the Nernst equation. Osmosis Across Selectively Permeable Mem- branes—“Net Diffusion” of Water (p. 53) Osmosis Is the Process of Net Movement of Water Caused by a Concentration Difference of Water. Water is the most abundant substance to diffuse through the cell membrane. However, the amount that diffuses in each direction is so precisely balanced under normal conditions that not even the slightest net movement of water molecules occurs. Therefore, the volume of a cell remains constant. However, a concentration difference for water can develop across a cell membrane. When this happens, net movement of water occurs across the 2 cell membrane, causing the cell to either swell or shrink, depending on the direction of the net movement. The pressure difference required to stop osmosis is the osmotic pressure. The Osmotic Pressure Exerted by Particles in a Solution Is Determined by the Number of Particles per Unit Volume of Fluid and Not by the Mass of the Particles. On average, the kinetic energy of each molecule or ion that strikes a membrane is about the same regardless of its molecular size. Consequently, the factor that determines the osmotic pressure of a solution is the concentration of the solution in terms of number of particles per unit volume but not in terms of the mass of the solute. The Osmole Expresses Concentration in Terms of Number of Particles. One osmole is 1 gram molecular weight of undissociated solute. Thus, 180 grams of glucose, which is 1 gram molecular weight of glucose, is equal to 1 osmole of glucose because glucose does not dissociate. A solution that has 1 osmole of solute dissolved in each kilogram of water is said to have an osmolality of 1 osmole per kilogram, and a solution that has 1/1000 osmole dissolved per kilogram has an osmolality of 1 milliosmole per kilogram. The normal /fluids is osmolality of the extracellular and intracellular c about 300 milliosmoles per kilogram, and the osmotic pressure of these fluids is about 5500 mm Hg. 36 UNIT II Membrane Physiology, Nerve, and Muscle The Na+-K+ Pump Controls Cell Volume. The Na+-K+ pump transports three molecules of sodium to the outside of the cell for every two molecules of potassium pumped to the inside. This continual net loss of ions from the cell interior initiates an osmotic force to move water out of the cell. Furthermore, when the cell begins to swell, the Na+-K+ pump is automatically activated, moving to the exterior still more ions that are carrying water with them. Therefore, the Na+-K+ pump performs a continual surveillance role in maintaining normal cell volume. Active Transport Saturates in the Same Way That Facilitated Diffusion Saturates. When the difference in concentration of the substance to be transported is small, the rate of transport rises approximately in proportion to the increase in concentration. At high concentrations, the rate of transport is limited by the rates at which the chemical reactions of binding, release, and protein carrier conformational changes can occur. Co-Transport and Counter-Transport Are Two Forms of Secondary Active Transport. When sodium ions are transported out of cells by primary active transport, a large concentration gradient of sodium normally develops. This gradient represents a storehouse of energy because the excess sodium outside the cell membrane is always attempting to diffuse to the cell interior. Co-transport. The diffusion energy of sodium can pull other substances along with the sodium (in the same direction) through the cell membrane using a special carrier protein. Counter-transport. The sodium ion and substance to be counter-transported move to opposite sides of the membrane, with sodium always moving to the cell interior. Here again, a protein carrier is required. Glucose and Amino Acids Can Be Transported Into Most Cells by Sodium Co-Transport. Transport carrier proteins have two binding sites on their exterior side—one for sodium and one for glucose or amino acids. Again, the concentration of sodium ions is relatively high on the outside and relatively low on the inside, providing the energy for the transport. A special property of transport proteins is that the conformational change that allows sodium movement to the cell interior does not occur until a glucose or amino acid molecule also attaches to its specific protein carrier. Calcium and Hydrogen Ions Can Be Transported Out of Cells Through the Sodium Counter-Transport Mechanism. Calcium counter-transport occurs in most cell mem- branes, with sodium ions-> moving to the cell interior Transport of Substances Through Cell Membranes 37 and calcium ions - moving > to the exterior; both are bound to the same transport protein in a counter- transport mode. Hydrogen counter-transport occurs especially in the proximal tubules of the kidneys, where sodium ions move from the lumen of the tubule to the interior of the tubular cells, and hydrogen ions are counter- transported into the lumen. CHAPTER 5 Membrane Potentials and Action Potentials Electrical potentials exist across the membranes of essentially all cells of the body. In addition, nerve and muscle cells are “excitable,” which means they are capable of self-generating electrical impulses at their membranes. The present discussion is concerned with membrane potentials that are generated both at rest and during action potentials by nerve and muscle cells. BASIC PHYSICS OF MEMBRANE POTENTIALS (p. 61) A Concentration Difference of Ions Across a Selectively Permeable Membrane Can Produce a Membrane Potential. Potassium diffusion potential. The neuronal cell membrane is highly permeable to potassium ions compared with most other ions. Potassium ions tend to diffuse outward because of their high concentration inside the cell. Because potassium ions are positively charged, the loss of potassium ions from the cell creates a negative potential in- side the cell. This negative membrane potential is sufficiently great to block further net diffusion of potassium despite the high potassium ion concen- tration gradient. In the normal large mammalian nerve fiber, the potential difference required to stop further net diffusion of potassium is about −94 millivolts. Sodium diffusion potential. Now let us imagine that a cell membrane is permeable to sodium ions but not to any other ions. Sodium ions would diffuse into the cell because of the high sodium concentration out- side the cell. The diffusion of sodium ions into the cell would create a positive potential inside the cell. Within milliseconds the membrane potential would rise to a sufficiently high level to block further net diffusion of sodium ions into the cell. This poten- tial is about +61 millivolts for the large mammalian nerve fiber. The Nernst Equation Describes the Relation of Diffusion Potential to Concentration Difference. The membrane potential that prevents net diffusion of an ion in either direction through the membrane is called the 38 Membrane Potentials and Action Potentials 39 Nernst potential for that ion. The Nernst equation is as follows: Concentration inside EMF millivolts 61 × log z Concentration outside where EMF is the electromotive force in millivolts and z is the electrical charge of the ion (e.g., +1 for K+). The sign of the potential is positive (+) if the ion under consideration is a negative ion and negative (−) if it is a positive ion. The Goldman Equation Is Used to Calculate the Diffusion Potential When the Membrane Is Permeable to Several Different Ions. When the membrane is permeable to several different ions, the diffusion potential that develops depends on three factors: (1) the polarity of the electrical charge of each ion, (2) the permeability of the membrane (P) to each ion, and (3) the concentrations (C) of the respective ions on the inside (i) and outside (o) of the membrane. The Goldman equation is as follows: CNai PNa C Ki P K C Cl o P Cl EMF millivolts 61 × log CNao PNa CK oPK C Cl i P Cl Note the following features and implications of the Goldman equation: Sodium, potassium, and chloride ions are most im- portantly involved in the development of membrane potentials in neurons and muscle fibers, as well as in the neuronal cells in the central nervous system. The degree of importance of each ion in determining the voltage is proportional to the membrane perme- ability for that particular ion. A positive ion concentration gradient from inside the membrane to the outside causes electronegativ- ity inside the membrane. RESTING MEMBRANE POTENTIAL OF NEURONS (p. 63) The Resting Membrane Potential Is Established by the Diffusion Potentials, Membrane Permeability, and Electrogenic Nature of the Sodium-Potassium Pump. Potassium diffusion potential. A high ratio of potas- sium ions from inside to outside the cell, 35:1, pro- duces a Nernst potential of −94 millivolts according to the Nernst equation. Sodium diffusion potential. The ratio of sodium ions from inside to outside the membrane is 0.1, which yields a calculated Nernst potential of +61 millivolts. Membrane permeability. The permeability of the nerve fiber membrane to potassium is about 100 times 40 UNIT II Membrane Physiology, Nerve, and Muscle greater compared with sodium, so the diffusion of potassium contributes far more to the membrane potential. This high value of potassium permeability in the Goldman equation yields an internal mem- brane potential of −86 millivolts, which is close to the potassium diffusion potential of −94 millivolts. Electrogenic nature of the sodium-potassium (Na+- K+) pump. The Na+-K+ pump transports three so- dium ions to the outside of the cell for each two potassium ions pumped to the inside, which causes a continual loss of positive charges from inside the membrane. Therefore, the Na+-K+ pump is electro- genic because it produces a net deficit of positive ions inside the cell, which causes a negative charge of about −4 millivolts inside the cell membrane. NEURON ACTION POTENTIAL (p. 65) Neuronal signals are transmitted by action potentials, which are rapid changes in membrane potential. Each action potential begins with a sudden change from the normal resting negative potential to a positive mem- brane potential and then ends with an almost equally rapid change back to the resting negative potential. The successive stages of the action potential are as follows: Resting stage. This is the resting membrane potential before the action potential occurs. Depolarization stage. At this time, the membrane suddenly becomes permeable to sodium ions, allow- ing tremendous numbers of positively charged so- dium ions to move to the interior of the axon. This movement of sodium ions causes the membrane potential to rise rapidly in the positive direction. Repolarization stage. Within a few ten-thousandths of a second after the membrane becomes highly per- meable to sodium ions, the voltage-gated sodium channels begin to close and the voltage-gated potas- sium channels begin to open. Then rapid diffusion of potassium ions to the exterior re-establishes the normal negative resting membrane potential. Voltage-Gated Sodium and Potassium Channels Are Activated and Inactivated During the Course of an Action Potential. The voltage-gated sodium channel is necessary for both depolarization and repolarization of the neuronal membrane during an action potential. The voltage-gated potassium channel also plays an important role in increasing the rapidity of repolarization of the membrane. These two voltage-gated channels are present Membrane Potentials and Action Potentials 41 in addition to the Na+-K+ pump and the Na+-K+ leak channels that establish the resting permeability of the membrane. Summary of the Events That Cause the Action Potential. During the resting state, before the action poten- tial begins, the conductance for potassium ions is about 100 times as great as the conductance for so- dium ions. This is caused by much greater leakage of potassium ions than sodium ions through the leak channels. At the onset of the action potential, the voltage-gated sodium channels instantaneously become activated and allow up to a 5000-fold increase in sodium per- meability (also called sodium conductance). The in- activation process then closes the sodium channels within a few fractions of a millisecond. The onset of the action potential also causes voltage gating of the potassium channels, causing them to begin opening more slowly. At the end of the action potential, the return of the membrane potential to the negative state causes the potassium channels to close back to their original status but, again, only after a delay. A Positive-Feedback, Vicious Cycle Opens the Sodium Channels. If any event causes the membrane potential to rise from −90 millivolts toward the zero level, the rising voltage itself causes many voltage-gated sodium channels to begin opening. This action allows rapid inflow of sodium ions, which causes still further rise of the membrane potential, thus opening still more voltage-gated sodium channels. This process is a positive-feedback vicious cycle that continues until all of the voltage-gated sodium channels have become activated (opened). An Action Potential Does Not Occur Until the Threshold Potential Has Been Reached. The threshold potential has been reached when the number of sodium ions entering the nerve fiber becomes greater than the number of potassium ions leaving the fiber. A sudden increase in the membrane potential in a large nerve fiber from −90 millivolts to about −65 millivolts usually causes explosive development of the action potential. This level of −65 millivolts is said to be the threshold of the membrane for stimulation. A New Action Potential Cannot Occur When the Membrane Is Still Depolarized From the Preceding Action Potential. Shortly after the action potential is initiated, the sodium channels become inactivated, and any amount of excitatory signal applied to these channels 42 UNIT II Membrane Physiology, Nerve, and Muscle at this point does not open the inactivation gates. The only condition that can reopen them is when the membrane potential returns either to or almost to the original resting membrane potential. Then, within another small fraction of a second, the inactivation gates of the channels open, and a new action potential can be initiated. Absolute refractory period. An action potential can- not be elicited during the absolute refractory period, even with a strong stimulus. This period for large myelinated nerve fibers is about 1/2500 second, which means that a maximum of about 2500 impuls- es can be transmitted per second. Relative refractory period. This period follows the absolute refractory period. During this time, stron- ger than normal stimuli are required to excite the nerve fiber and for an action potential to be initiated. PROPAGATION OF THE ACTION POTENTIAL (p. 69) An action potential elicited at any one point on a membrane usually excites adjacent portions of the membrane, resulting in propagation of the action potential. Thus, the depolarization process travels along the entire extent of the nerve fiber. Transmission of the depolarization process along a neuron or muscle fiber is called a neuronal or muscle impulse. Direction of propagation. An excitable membrane has no single direction of propagation; instead, the action potential travels in both directions away from the stimulus. Chemical synapses dictate directional- ity of action potentials. All-or-nothing principle. Once an action potential has been elicited at any point on the membrane of a normal fiber, the depolarization process travels over the entire membrane under normal conditions, or it might not travel at all if conditions are not normal. RE-ESTABLISHING SODIUM AND POTASSIUM IONIC GRADIENTS AFTER ACTION POTENTIALS ARE COMPLETED—IMPORTANCE OF ENERGY METABOLISM (p. 69) Transmission of each impulse along the nerve fiber reduces infinitesimally the concentration differences of sodium and potassium between the inside and outside of the membrane. From 100,000 to 50 million impulses can be transmitted by nerve fibers before the ion con- centration differences have decreased to the point that Membrane Potentials and Action Potentials 43 action potentials cannot occur. Even so, with time it becomes necessary to re-establish the sodium and potassium concentration differences across the mem- brane, which is achieved by the Na+-K+ pump. SPECIAL CHARACTERISTICS OF SIGNAL TRANSMISSION IN NERVE TRUNKS (p. 71) Large Nerve Fibers Are Myelinated and Small Ones Are Unmyelinated. The central core of the fiber is the axon, 2 and the membrane of the axon is used for conducting the action potential. Surrounding the larger axons is a thick myelin sheath deposited by Schwann cells. The sheath consists of multiple layers of cellular membrane containing the lipid substance sphingomyelin, which < is an excellent insulator. At the juncture between two successive Schwann cells, a small noninsulated area only 2 to 3 micrometers in length remains where ions can still flow with ease between the extracellular fluid and the axon interior. This area is the node of Ranvier. “Saltatory” Conduction Occurs in Myelinated Fibers. Even though ions cannot flow significantly through the thick sheaths of myelinated neurons, they can flow with considerable ease through the nodes of Ranvier. Thus, the neuronal impulse jumps from node to node along the fiber, which is the origin of the term “saltatory.” Saltatory conduction is of value for two reasons: Increased velocity. By causing the depolarization process to jump long intervals (up to about 1.5 milli- meters) along the axis of the nerve fiber, this mecha- nism increases the velocity of neuronal transmission in myelinated fibers as much as 5- to 50-fold. Energy conservation. Saltatory conduction conserves energy for the axon because only the nodes depolarize, allowing perhaps a hundred times smaller movement of ions than would otherwise be necessary and there- fore requiring little energy for re-establishing the so- dium and potassium concentration differences across the membrane after a series of neuronal impulses. Conduction Velocity Is Greatest in Large, Myelinated Nerve Fibers. The velocity of action potential conduction in nerve fibers varies from as low as 0.25 m/sec in very small unmyelinated fibers to as high as 100 m/sec in very large myelinated fibers. The velocity increases approximately with the fiber diameter in myelinated nerve fibers and approximately with the square root of the fiber diameter in unmyelinated fibers. CHAPTER 6 Contraction of Skeletal Muscle About 40 percent of the body mass is skeletal muscle, and perhaps another 10 percent is smooth muscle and cardiac muscle. Many of the principles of contraction apply to all three types of muscle. In this chapter, the function of skeletal muscle is considered. The functions of smooth muscle are discussed in Chapter 8, and the functions of cardiac muscle are discussed in Chapter 9. PHYSIOLOGICAL ANATOMY OF SKELETAL MUSCLE (p. 75) Skeletal Muscle Fiber Figure 6–1 shows the organization of skeletal muscle. In most muscles, the fibers extend the entire length of the muscle. Each fiber is innervated by only one nerve ending. Myofibrils Are Composed of Actin and Myosin Filaments. Each muscle fiber contains hundreds to thousands of myofibrils; in turn, each myofibril (see Figure 6–1D) is composed of about 1500 myosin filaments and 3000 actin filaments lying side by side. These filaments are large polymerized protein molecules that are responsible for muscle contraction. In Figure 6–1 the thick filaments are myosin, and the thin filaments are actin. Note the following features: Light and dark bands. The myosin and actin filaments partially interdigitate and thus cause the myofibrils to have alternate light and dark bands. The light bands contain only actin filaments and are called I bands. The dark bands, called A bands, contain myosin filaments as well as the ends of the actin filaments. The length of the A band is the length of the myosin filament. The length of the I band changes with muscle contraction. Cross-bridges. The small projections from the sides of the myosin filaments are cross-bridges. They protrude from the surfaces of the myosin filament along its en- tire length except in the center. Myosin cross-bridges interact with actin filaments, causing contraction. Z disk. The ends of the actin filaments are attached to Z disks (see Figure 6–1E). The Z disk passes across the myofibril and from one to another, attaching and align- ing the myofibrils across the muscle fiber. The entire muscle fiber therefore has light and dark bands, giving skeletal and cardiac muscle a striated appearance. 44 Contraction of Skeletal Muscle 45 SKELETAL MUSCLE A Muscle B C Muscle fasciculus Z disk A I band band Myofibril Z A I Muscle fiber disk band band D H band Z Sarcomere Z G-Actin molecules E H J Myofilaments F-Actin filament K L Myosin filament Myosin molecule M F G H I N Light Heavy meromyosin meromyosin Figure 6–1 Organization of skeletal muscle, from the gross to the mo- lecular level. F, G, H, and I are cross sections at the levels indicated. Sarcomere. The portion of a myofibril that lies be- tween two successive Z disks is called a sarcomere. During rest, the actin filaments overlap the myosin filaments with an optimal amount of interdigitation in skeletal muscle and slightly shorter than optimal interdigitation in cardiac muscle. GENERAL MECHANISM OF MUSCLE CONTRACTION (p. 77) The initiation and execution of muscle contraction occur in the following sequential steps: 1. An action potential travels along a motor neuron to its endings on muscle fibers, and each neuronal 46 UNIT II Membrane Physiology, Nerve, and Muscle ending secretes a small amount of the neurotrans- mitter substance acetylcholine. 2. The acetylcholine diffuses to a local area of the mus- cle membrane, causing acetylcholine-gated cation channels to open. Sodium, potassium, and calcium ions move through the cation channels down their individual electrochemical gradients. The net effect is development of a local depolarization, called a generator potential or end-plate potential. The local depolarization in turn leads to opening of voltage- gated sodium channels in the muscle membrane. A muscle fiber action potential follows. 3. The action potential travels along the muscle fiber membrane, causing the sarcoplasmic reticulum to release calcium ions into the sarcoplasm. 4. The calcium ions initiate attractive forces between the actin and myosin filaments of the myofibrils, causing them to slide together, which is the contractile process. 5. The calcium ions are continually pumped back into the sarcoplasmic reticulum where they remain stored until a muscle action potential arrives; this removal of calcium ions from the sarcoplasm causes muscle contraction to cease. MOLECULAR MECHANISM OF MUSCLE CONTRACTION (p. 78) Muscle Contraction Occurs by a Sliding Filament Mechanism. Mechanical forces generated by interactions between actin and myosin filaments cause the actin filaments to slide inward among the myosin filaments. Under resting conditions, these forces are inhibited, but when an action potential travels over the muscle fiber membrane, the sarcoplasmic reticulum releases large quantities of calcium ions, which activate the forces between the myosin and actin filaments, causing contraction to begin. Myosin Filaments Are Composed of Multiple Myosin Molecules. The tails of myosin molecules bundle together to form the body of the filament, whereas the myosin heads and part of each myosin molecule hang outward to the sides of the body, providing an arm that extends the head outward from the body. The protruding arms and heads together are called cross- bridges. An important feature of the myosin head is that - it functions as an adenosine triphosphatase enzyme, which allows it to cleave adenosine triphosphate (ATP) and thus energize the contraction process. Actin Filaments Are Composed of Actin, Tropomyosin, and Troponin. Each actin filament is about 1 micrometer long. Contraction of Skeletal Muscle 47 The bases of the actin filaments are inserted strongly into the Z disks, whereas the other ends protrude in both directions into the adjacent sarcomeres where they lie in the spaces between the myosin molecules. Interaction of One Myosin Filament, Two Actin Filaments, and Calcium Ions to Cause Contraction (p. 79) The actin filament is inhibited by the troponin- tropomyosin complex. Activation is stimulated by calcium ions. Inhibition by the troponin-tropomyosin complex. The active sites on the normal actin filament of the relaxed muscle are inhibited or physically covered by the tro- ponin-tropomyosin complex. Consequently, the sites cannot attach to the heads of the myosin filaments to cause contraction until the inhibitory effect of the troponin-tropomyosin complex is itself inhibited. Activation by calcium ions. The inhibitory effect of the troponin-tropomyosin complex on the actin fila- ments is inhibited in the presence of calcium ions. Calcium ions combine with troponin C, causing the troponin complex to tug on the tropomyosin mol- ecule. This action “uncovers” the active sites of the actin, allowing myosin heads to attach and contrac- tion to proceed. A “Walk-Along” Theory Can Explain How the Activated Actin Filament and the Myosin Cross-Bridges Interact to Cause Contraction. When a myosin head attaches to an active site, the head tilts automatically toward the arm that is dragging along the actin filament. This tilt of the head is called the power stroke. Immediately after tilting, the head automatically breaks away from the active site. The head then returns to its normal perpendicular direction. In this position, it combines with a new active site farther along the actin filament. Thus, the heads of the cross-bridges bend back and forth and, step by step, walk along the actin filament, pulling the ends of the actin filaments toward the center of the myosin filament. The Amount of Actin and Myosin Filament Overlap Determines Tension Developed by the Contracting Muscle (p. 81) The Strength of Contraction Is Maximal When There Is Optimal Overlap Between Actin Filaments and the Cross- Bridges of the Myosin Filaments. A muscle cannot develop tension at very long, nonphysiological sarcomere lengths 48 UNIT II Membrane Physiology, Nerve, and Muscle because there is no overlap between actin and myosin filaments. As the sarcomere shortens and actin and myosin filaments begin to overlap, the tension increases progressively. Full tension is maintained at a sarcomere length of about 2.0 micrometers because the actin filament has overlapped all of the cross-bridges of the myosin filament. Upon further shortening, the ends of the two actin filaments begin to overlap (in addition to overlapping the myosin filaments), causing muscle tension to decrease. When the sarcomere length decreases to about 1.65 micrometers, the two Z disks of the sarcomere abut the ends of the myosin filaments, and the strength of contraction decreases greatly. ENERGETICS OF MUSCLE CONTRACTION (p. 82) Muscle Contraction Requires ATP to Perform Three Main Functions Most of the ATP is used to activate the walk-along mechanism of muscle contraction. Active transport of calcium ions back into the sarco- plasmic reticulum causes contraction to terminate. Active transport of sodium and potassium ions through the muscle fiber membrane maintains an appropriate ionic environment for the propagation of action potentials. There Are Three Main Sources of Energy for Muscle Contraction. The concentration of ATP in the muscle fiber is sufficient to maintain full contraction for only 1 to 2 seconds. After the ATP is split into adenosine diphosphate (ADP), the ADP is rephosphorylated to form a new ATP. There are several sources of energy for this rephosphorylation. Phosphocreatine carries a high-energy bond similar to that of ATP but has more free energy. The energy released from this bond causes bonding of a new inorganic phosphate ion to ADP to reconstitute the ATP. The combined energy of ATP and phosphocre- atine is capable of causing maximal muscle contrac- tion for only 5 to 8 seconds. The breakdown of glycogen to pyruvic acid and lactic acid liberates energy that is used to convert ADP to ATP. The glycolytic reactions can occur in the ab- sence of oxygen. The rate of formation of ATP by the glycolytic process is about 2.5 times as rapid as ATP formation when the cellular foodstuffs react with ox- ygen. Glycolysis alone can sustain maximum muscle contraction for only about 1 minute. Contraction of Skeletal Muscle 49 Oxidative metabolism occurs when oxygen is com- bined with the various cellular foodstuffs to liberate ATP. More than 95 percent of all energy used by the muscles for sustained, long-term contraction is de- rived from this source. The foodstuffs consumed are carbohydrates, fats, and proteins. CHARACTERISTICS OF WHOLE MUSCLE CONTRACTION (p. 83) Isomet