21BTC204 Unit II - Final PDF
Document Details
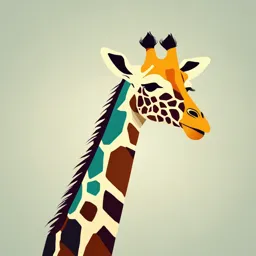
Uploaded by PermissibleMossAgate2914
SRM University
Tags
Summary
This document covers the fundamentals of bioreaction, fermentation, and kinetics. It describes the different types of bioreactors and phases of microbial growth, including the lag phase, log phase, stationary phase, and death phase. The document also includes information on growth kinetics and models, as well as growth inhibitors.
Full Transcript
UNIT -II Bioreaction Any reaction that involves biological molecule to obtain the desired end product is known to be a bioreaction. It can be a reaction that takes place inside the bioreactor Example: The metabolic growth of bacteria inside the fermenter by utilizing the sub...
UNIT -II Bioreaction Any reaction that involves biological molecule to obtain the desired end product is known to be a bioreaction. It can be a reaction that takes place inside the bioreactor Example: The metabolic growth of bacteria inside the fermenter by utilizing the substrate and producing the product of interest. Components of a Bioreactor Different type of bioreactors: (a) a stirred tank for microbial culture, (b) a stirred tank for cell culture, (c) an air-lift fermenter, and (d) a hollow-fiber bioreactor. Fermentation Kinetics Kinetics is a branch of chemistry that specifically focuses on the rates of chemical reactions. It aims to understand the chemical reaction rate dynamics and mechanisms. How the reactants transform into products as their molecules collide and their chemical bonds break and form -The pathways they thread, and -The energy that drives the transformation -Kinetics speaks about reaction rates rooted in mathematical analysis and experimental observations. -Kinetics studies helps us to understand how quickly or slowly chemical reactions occur? What could have speeded them up or slowed them down. Fundamental Concepts in Kinetics Reaction rate laws (or rate equations): The mathematical expressions describing the relationship between chemical reaction rate(s) and reactant concentration(s). The rate laws include the reaction rate constant and the reaction orders. Reaction rate constant: The proportionality constant specific to the reaction. Reaction orders: The mathematical relationship between the reaction rate and the concentration(s) of reactant(s), indicating how reactant concentration(s) influence the rate of a chemical reaction. The common reaction orders are zero-order, first-order, and second-order reactions. Growth Curve In a closed system with enough nutrients, a bacteria shows a predictable growth pattern that is the bacterial growth curve. It consists of four different phases. Phases of the Bacterial Growth Curve Upon inoculation into a new nutrient medium, the bacteria shows four distinct phases of growth. Let us dive into each of the phases in detail – Lag Phase The bacteria upon introduction into the nutrient medium take some time to adapt to the new environment. In this phase, the bacteria does not reproduce but prepares itself for reproduction. The cells are active metabolically and keep increasing in size. The cells synthesize RNA, growth factors and other molecules required for cell division. Log Phase The bacterial cells enter the log phase soon after the lag phase, i.e., the preparation phase. The log phase is also known as the exponential phase. The doubling of the bacterial cells marks this phase. The cell number increases in a logarithmic fashion such that the cell constituent is maintained. The log phase continues until there is depletion of nutrients in the setup. The stage also comes to a stop if toxic substances start to accumulate, resulting in a slower growth rate. Stationary Phase In the stationary phase, the rate of growth of the cells becomes equal to its rate of death. The rate of growth of the bacterial cells is limited by the accumulation of toxic compounds and also depletion of nutrients in the media. The cell population remains constant at this stage. Plotting this phase on the graph gives a smooth horizontal linear line. Death Phase This is the last phase of the bacterial growth. At this stage, the rate of death is greater than the rate of formation of new cells. Lack of nutrients, physical conditions or other injuries to the cell leads to death of the cells Growth phase of bacterial cell Determining the kinetics of cell development between divisions is extremely difficult because the parameters for measuring growth (such as dry mass, volume, and linear dimensions) do not behave consistently even within the same cell. Studies of both fixed and living cells have revealed two distinct growth patterns: linear and exponential. A pattern of exponential growth indicates that the growth rate is proportional to the total mass; as the mass grows, so does the growth rate. The growth rate is proportional to the amount of active protoplasm or replicating organisms. A linear growth pattern indicates that the growth rate remains constant and does not rise during the cell cycle. In this case, growth rate is independent of cell mass and is instead proportional to a constant number of elements (synthetic sites) whose activities remain constant during the growth cycle. Quantifying growth kinetics Unstructured Nonsegregated Models to Predict Specific Growth Rate Substrate limited growth Models with growth inhibitors The inhibition pattern of microbial growth is analogous to enzyme inhibition. Substrate inhibition Product inhibition Inhibition by toxic compound Example : Cell growth in ethanol fermentation Bacterial cell growth parameters Optimization of bacterial cell growth environment A) TEMPERATURE: Bacteria have a minimum, optimum, and maximum temperature for growth and can be divided into 3 groups based on their optimum growth temperature: 1. Psychrophiles are cold-loving bacteria. Their optimum growth temperature is between -5C and 15C. They are usually found in the Arctic and Antarctic regions and in streams fed by glaciers. 2. Mesophiles are bacteria that grow best at moderate temperatures. Their optimum growth temperature is between 25C and 45C. Most bacteria are mesophilic and include common soil bacteria and bacteria that live in and on the body. 3. Thermophiles are heat-loving bacteria. Their optimum growth temperature is between 45C and 70C and are commonly found in hot springs and in compost heaps. 4. Hyperthermophiles are bacteria that grow at very high temperatures. Their optimum growth temperature is between 70C and 110C. They are usually members of the Archaea and are found growing near hydrothermal vents at great depths in the ocean. B) OXYGEN REQUIREMENTS Bacteria show a great deal of variation in their requirements for gaseous oxygen. Most can be placed in one of the following groups: 1. Obligate aerobes are organisms that grow only in the presence of oxygen. They obtain their energy through aerobic respiration. 2. Microaerophils are organisms that require a low concentration of oxygen (2% to 10%) for growth, but higher concentrations are inhibitory. They obtain their energy through aerobic respiration. Obligate anaerobes are organisms that grow only in the absence of oxygen and, in fact, are often inhibited or killed by its presence. They obtain their energy through anaerobic respiration or fermentation. Aerotolerant anaerobes , like obligate anaerobes, cannot use oxygen to transform energy but can grow in its presence. They obtain energy only by fermentation and are known as obligate fermenters. Facultative anaerobes are organisms that grow with or without oxygen, but generally better with oxygen. They obtain their energy through aerobic respiration if oxygen is present but use fermentation or anaerobic respiration if it is absent. Most bacteria are facultative anaerobes. pH Microorganisms can be placed in one of the following groups based on their optimum pH requirements: 1. Neutrophiles grow best at a pH range of 5 to 8. 2. Acidophiles grow best at a pH below 5.5. 3. Alkaliphiles grow best at a pH above 8.5. OSMOSIS Osmosis is the diffusion of water across a membrane from an area of higher water concentration (lower solute concentration) to lower water concentration (higher solute concentration). Osmosis is powered by the potential energy of a concentration gradient and does not require the expenditure of metabolic energy. Most bacteria require an isotonic environment or a hypotonic environment for optimum growth. Organisms that can grow at relatively high salt concentrations (up to 10%) are said to be osmotolerant. Those that require relatively high salt concentrations for growth, like some of the Archaea that require sodium chloride concentrations of 20 % or higher halophiles. Nutritional requirements In addition to a proper physical environment, microorganisms also depend on an available source of chemical nutrients. Microorganisms are often grouped according to their energy source and their source of carbon. ENERGY SOURCE 1. Phototrophs use radiant energy (light) as their primary energy source. 2. Chemotrophs use the oxidation and reduction of chemical compounds as their primary energy source. Carbon is the structural backbone of the organic compounds that make up a living cell. Based on their source of carbon bacteria can be classified as autotrophs or heterotrophs. Nitrogen is needed for the synthesis of such molecules as amino acids, DNA, RNA and ATP. Depending on the organism, nitrogen, nitrates, ammonia, or organic nitrogen compounds may be used as a nitrogen source. 1. Sulfur - Sulfur is needed to synthesizes sulfur-containing amino acids and certain vitamins. Depending on the organism, sulfates, hydrogen sulfide, or sulfur-containing amino acids may be used as a sulfur source. 2. Phosphorus - Phosphorus is needed to synthesize phospholipids , DNA, RNA, and ATP. Phosphate ions are the primary source of phosphorus. 3. Potassium, magnesium, and calcium - These are required for certain enzymes to function as well as additional functions. 4. Iron - Iron is a part of certain enzymes. 5. Trace elements - Trace elements are elements required in very minute amounts, and like potassium, magnesium, calcium, and iron, they usually function as cofactors in enzyme reactions. They include sodium, zinc, copper,molybdenum, manganese, and cobalt ions. Cofactors usually function as electron donors or electron acceptors during enzyme reactions. E. WATER F. GROWTH FACTORS Growth factors are organic compounds such as amino acids , purines , pyrimidines , and vitamins that a cell must have for growth but cannot synthesize itself. Organisms having complex nutritional requirements and needing many growth factors are said to be fastidious. Criteria for good medium It will produce the maximum yield of product or biomass per gram of substrate used. It will produce the maximum concentration of biomass or product. It will permit the maximum rate of product formation. There will be a minimum yield of undesired products. It will be of consistent quality and available throughout the year. It will cause minimal problems during medium sterilization, other aspects of the production process such as aeration, agitation, downstream processing, waste treatment. Medium designed will affect the design of fermenter, oxidation of hydrocarbons highly aerobic process –air lift reactor. Problems will be encountered in scaling up. Since large reactors will have low mass transfer rate. High viscous medium will consume more power. Besides growth and product formation medium will influence the pH variation, foam formation, morphological form of organism etc., Use of complex nutrients will influence downstream processing Variation in complex nutrients will result in batch-to-batch variations. Medium cost has to be considered depending on the product type. E.g. For single-cell protein production, the medium cost is more than 50 % of the production cost. Medium formulation Medium formulation is essential stage in manufacturing process Carbon & Nitrogen other Energy + sources + O2 + nutrients Sources Biomass + products + CO2 +H2O +heat Elemental composition of microorganisms may be taken as guide Design of medium will influence the oxygen requirements Nutrient sources for industrial fermentation Nutrient Raw material ❑ Carbon source Glucose Corn sugar, Starch, Cellulose Sucrose Sugarcane, Sugar beet molasses Lactose Milk whey Fats Vegetable oils Hydrocarbons Petroleum fractions ❑ Nitrogen source Protein Soybean meal, Corn steep liquor, Distillers' solubles Ammonia Pure ammonia or ammonium salts Nitrate Nitrate salts Nitrogen Air ❑ Phosphorous source Phosphate salts Energy Sources Energy comes either from oxidation of medium components or from light. Most industrial microorganism are chemo-organotrophs, therefore the commonest source of energy will be the carbon (CHO), lipids & protein. Some micro-organisms can also use hydrocarbons or methanol as carbon & energy sources. Factors influencing the carbon source - Cost of the product - rate at which it is metabolized - geographical locations - government regulations - cellular yield coefficient Methane - 0.62 Alkanes - 1.03 Glucose - 0.51 Acetate - 0.34 Examples of carbon sources Carbohydrates Starch – max 2% Molasses Sucrose Glucose Malt (Barley grains germinated and heat treated) Molasses would normally be used as the cheapest carbohydrate to grow yeast biomass in a large scale process. But this is not acceptable for Recombinant protein production b/c of difficulties and cost for purification. C has a dual role in Biosynthesis & energy generation. C requirement for biomass production under aerobic condition may be estimated the cellular yield Y which is defined as, Quantity of cell dry matter produced/quantity of C substrate utilized. Oils and fats Oils are first used as antifoams and later used as carbon sources (soya oil, olive oil, maize oil, linseed oil etc.,) Factors favoring oil 2.4 times energy than glucose Hence volume advantage of 4 times. some organisms can use only oils for efficient production Eg. antibiotics (Methyl oleate is used in cephalosporin) Nitrogen sources Inorganic Ammonia gas, ammonium chloride, ammonium sulphate, ammonium nitrates, sodiumnitrates Ammonia gas used for pH control Ammonium salts produces acid conditions when ammonia is utilized. pH drift Sodium nitrate produces alkaline drift. Organic nitrogen may be supplied by amino acids, protein, urea Growth will be faster. These are commonly added as complex nitrogen sources such as soy bean meal, corn steep liquor etc., (During storage these sources are affected by moisture, temperature and ageing) Elemental composition Element Bacteria Yeast Fungi Carbon 50-53 45-50 40-63 Hydrogen 7 7 7 Nitrogen 12-15 7.5-11 7-10 Phosphorus 2-3 0.8-2.6 0.4-4.5 Sulphur 0.2-1.0 0.01-0.24 0.1-0.5 Potassium 1.0-4.5 1-4 0.2-2.5 Sodium 0.5-1.0 0.01-0.1 0.02-0.5 Calcium 0.01-1.1 0.1-0.3 0.1-1.4 Magnesium 0.1-0.5 0.1-0.5 0.1-0.5 Chloride 0.5 -- -- Iron 0.02-0.2 0.01-0.5 0.1-0.2 WATER Assessing suitability of water - pH - dissolved salts - effluent contamination In olden days mineral content is important - High Ca for dark beers - High carbonate for stouts Nowadays - Deionization of water Reuse of water is important - It reduces water cost by 50% - Effluent treatment cost by 10 fold Nitrogen Sources - Organic 8% nitrogen: – Soybean meal. – Groundnut (peanut) meal. – Pharmamedia (cottonseed derived). 4.5% nitrogen: – Cornsteep powder – (maize derived). – Whey powder. 1.5-2% nitrogen: – Cereal flours. – Molasses. Minerals All microorganisms require minerals for growth, (metabolism) and product formation Magnesium, phosphorus, potassium, sulphur, calcium, chlorine are essential components Cobalt, copper, manganese, iron, molybdenum, zinc are also essential but in traces. Also, depending on product analysis apart from biomass minerals will be decided. E.g. sulphur in penicillin, cephalosporin, chlorine in chlortetracycline etc., P is used as a Buffer to minimize pH changes when external pH is not being used. Concentration of phosphate in the medium is normally required in excess for buffering the medium. Phosphate concentration in the medium are critical in antibiotic production since some enzymes of biosynthesis are influenced by phosphate. Chelators Many media cannot be prepared without precipitation during autoclaving. Hence some chelating agents are added to form complexes with metal ions which are gradually utilised by microorganism Examples of chelators:EDTA, citric acid, polyphosphates etc., It is important to check the concentration of chelators otherwise it may inhibit the growth. In many media these are added separately after autoclaving Or yeast extract, peptone complex with these metal ions. Growth Factors: Some microorganisms cannot synthesize a full complement of cell components and therefore require preformed compounds called growth factors Eg: vitamins, aminoacids, fatty acids or sterols Complex media sources contain most of these compounds. Careful blending of these will give the required growth factors. For vinegar production – Calcium Pantothenate For Glutamic acid – Biotin Buffers The control of pH may be extremely important if optimal productivity is to be achieved. Many media are buffered at about pH 7.0 by the incorporation of CaCO3, if pH decreases the CO3 is decomposed. PO4 which are the part of many media also play an important role in buffering. High PO4 conc. are critical in the production of many secondary metabolites. C & N sources will also a basis for pH control as buffering capacity can be provided by the protein, peptides & AA such as in corn steep liquor. The pH may also be controlled externally by addition of NH3 or NaOH & H2SO4. The addition of precursors & metabolic regulators to media Some components of a fermentation medium help to regulate the production of the product rather than support the growth of the micro-organism. Such additive include, precursors, inhibitors, Precursors: Some chemicals when added to certain fermentation are directly incorporated into the desired product. Eg: Improving the yields of Penicillin production A range of different side chain can be incorporated into the penicillin molecule, eg corn steep liquor increased the yield of penicillin form 20 units to 100 units. Corn steep liquor contain phenylethlamine when incorporated, it yield benzyl penicillin. Phenylacetic acid is widely used precursor in penicillin production. Inhibitors When certain inhibitors are added to fermentation more of a specific product may be produced.Eg : Glycerol fermentation Glycerol production depends on modifying ethanol fermentation by removing acetaldehyde. Addition of sodium bisulphite forms acetaldehyde bisulphite. Acetaldehyde is no longer available and dihydroxy acetone is formed. Inhibitors have also been used to affect cell wall structure and increase the permeability for release of metabolites. The best example is the use of penicillin & surfactants in glutamic acid production. Inducers Majority of the enzymes are inducible. Most inducers which are included in microbial enzyme media are substrate or substrate analogues but intermediates and products may sometime be used as inducers. Inducers are often substrate such as starch or dextrins for amylase, maltose for pullulanase & pectin for pectinase. E.g maltodextrins will induce amylase & fatty acids induce lipase. (use depend upon the cost) Antifoams Most fermentations foaming is major problem. It may be due to component in the medium or some factor produced by the microorganism. Foaming can be controlled by – Modification of medium – Mechanical foam breakers – Chemical agents antifoams are added Eg: Fatty acids, silicones, PPG 2000 Antifoams are surface active agents reducing the surface tension in the foam and destabilizing the protein films An ideal antifoam should have the following properties – Disperse readily and have fast action – Active at low concentrations – Long acting in preventing new foam – Should not be metabolized – Should not be toxic to microorganism and humans etc – Cheap, should not cause problem in fermentation Medium Can Be a Significant Proportion of Total Product Cost Elements of total product cost (%) Raw materials costs range from 38-77% in the examples shown Types of media Liquid media Solid and semi solid media Basic types of media on basis of composition A. Natural cultural media or empirical culture media Milk, diluted blood, vegetable juice, meat extracts. B. Synthetic or define culture media Dilute, reproducible solutions of chemically pure, known inorganic or organic compounds. C. Liquid culture media Living cells as tissue, or callus or organ uses of viruses, rickets etc. For eg. Chick embryo are commonly used for cultivation of viruses. Other types of media Simple or basal media Complex media Selective media Differential media Selective differential media Crude and Defined Media Media can be loosely assigned two types Defined media – Made from pure compounds Crude media – Made from complex mixtures (agricultural products) – Individual ingredients may supply more than one requirement – May contain polymers or even solids! Defined Media – Advantages Limitations Consistent Expensive – Composition Need to define and supply – Quality all growth factors…only Facilitate R and D mineral salts present Unlikely to cause foaming Yields and volumetric Easier upstream productivity can be poor: processing (formulation, – Cells have to “work sterilization etc.) harder”…proteins etc. are not Facilitate downstream present processing (purification – Missing growth etc.) factors…amino acids etc. Defined Media Main use is for low volume/high value-added products, especially proteins produced by recombinant organisms Some “defined” media may contain small amounts of undefined ingredients (e.g. yeast extract) to supply growth factors. Disadvantages Crude Media Variability: Cheap – Composition Provide growth factors – Quality (even “unknown” ones) – Supply Good yields and – Cost (Agri-politics) volumetric productivity Unwanted components-iron or copper which can often be lethal to cell growth May cause bioprocess foaming. Problems with upstream processing (medium pre-treatment and sterilization). Problems with downstream processing (product recovery and purification) Culture Media: (growth medium or culture medium is a liquid or gel designed to support the growth of microorganisms or cells. Natural and synthetic media Natural media It is the natural source of nutrients sufficient for the growth and proliferation of animal’s cells and tissue. There are three types Coagulans or plasma clots: available in the market in the form of liquid plasma kept in silicon ampoules or lyophilized plasma. (heparin). Biological fluid: serum from human adult blood, placental, cord blood, horse blood, calf blood or amniotic fluid, pleural fluid insect haemolymph serum etc. Tissue extract: such as embryo, liver spleen, leukocytes, tumor bone marrow etc Synthetic Media Prepared artificially by adding several nutrients (organic and inorganic), vitamins, salts, serum proteins, carbohydrates, cofactors, O2 and CO2 gas phases etc. Different type of synthetic media may be prepared for a variety of cells and tissue to cultured. It can be prepared for different functions. Basically, of two type Serum containing media and serum free media Serum free could be selective. Advantages and disadvantages of serum in culture medium Contains a complete set of essential growth factors, hormones etc. It binds and neutralizes the toxin. It contains protease inhibitors. It increases buffering capacity. Provides trace elements and other nutrients. Change from serum to serum-free media Serum can be reduced or omitted without apparent cell selection If appropriate nutritional and hormonal modifications were made to media. Disadvantages of Serum Not chemically defined May be the source of contamination Increase difficulties and cost downstream processing. Demand is increasing and can exceed supply Physiological Variability - Effects of albumin and transferrin are known Effects of nutrients (amino acids, nucleotides, sugars, etc), peptide growth factors, hormones, minerals and lipids on cell culture are unknown Shelf Life and Consistency - Serum varies from batch to batch - Might not last longer than a year - Should be replaced – might not be identical to first batch. Disadvantages of Serum Specificity - Several batches of serum should be held on reserve - Co culturing different cell types can be a problem Quality Control - Changing batch serum (replacement) requires extensive testing - Plating efficiency, Checking growth curve, Preservation of cell culture characteristics and Sterility. Contamination - Viruses and Mycoplasma - Country of origin and batch number Standardization - Standardization of experimental and production protocols is difficult Advantages of serum-free media Switch from growth enhancing medium for propagation to a differentiating inducing medium Regulate proliferation and differentiation Disadvantages of serum-free media Reagent purity – Degree of purity in reagents, water and cleanliness of apparatus needs to be high Cell proliferation – Growth is slower in serum free media Availability – of properly qualified-controlled serum-free media is limited - Products cultured are expensive Removal of serum also removes a source of proteins that provide a detoxifying action. Fewer generations can be obtained with serum free media – finite cell lines Multiplicity of media – Advantage to labs maintaining specific cell types but difficult for maintenance of many Selectivity – Some media may select a sublineage - Cells require different formulations Plant Culture Medium Plant Culture Medium Requirements Varies: – Plant cell type (woody, fern, orchid…) – Maintenance of callus or shoot formation (stage II) – Stimulation of Root and de-differentiation – Protoplast, suspension or batch cultures General Components: – Macronutrients, micronutrients, vitamins, amino acids, nitrogen, phosphorous, sugar, organic supplements and solidifying agents/support systems – AND growth regulators (hormones) Macronutrients (macro elements) - Needed in media in large amounts and make up ~0.1% of dry weight of plant: Nitrogen – supplied in form of ammonium ion (H4NO3+) and nitrate (KNO3) – best if both are present and together act to buffer pH. Some amino acids can supplement N requirements or take place as the N is removed via TCA and transglutaminases High ammonium causes a pale, glassy culture (vitrification) Potassium – come as counter ion with NO3-and PO4-2 Phosphorus – K2HPO4, H4NO3(HPO4)2, High concentrations of phosphate will lead to ppt with Ca+2. Micronutrients: Trace amount elements and salts necessary for growth: Fe (FeSO4) – Chelated to EDTA is most critical. The complex allows for a slow continuous release and avoids free metal generation of radical oxides after reaction with water. Others include: Zn, Cu, B, and Mo. Carbon and Energy Source – cultures do little if any photosynthesis (heterotrophs). Must supply carbon to metabolize ATP and other energy molecules. – Sucrose is usually used – Galactose, sorbitol and maltose also are used. Organic Supplements – Wide range of various needs Amino Acids – can provide nitrogen and support for metabolism as well as biosynthesis for new proteins, lipids and nucleotides Casine (milk protein) hydrolysates typically are the source of amino acids Vitamins: Vitamin B1 (thiamin) and Vitamin B6 (nicotinic acid pyridoxine), and myo-inositol. The latter is not a vitamin but used as one for plant culture media. Activated Charcoal (AC) –Used for it’s ability to bind hydrophobic compounds which inhibit growth. The actual role isn’t always clear nor is it always included in medium. Gelling Agents (support systems) – Solidified surface typically from the complex carbohydrates (non-digestible) extracted from seaweed (agar). Lots of variation between batches and suppliers Gums from plants, agarose can also be used Plant Culture Medium Growth Regulators- Five main classes; auxin, cytokinin, gibberellins, abscisic acid and ethylene. – Auxins- Promote cell division and growth – most auxins are synthetic and not found in plants. Naturally produced 1H-indol-3-acetic acid, is unstable to both heat and light. Naturally produced in apical and root meristems seeds and developing fruit Alters proton pump and ATP production in target cells Induces cell elongation Suppresses lateral bud growth and stimulates adventitious roots Synthetic form(s) include 2-4 dichorophenoxyacetic acid (2-4D) Acts as a herbicide by inducing unsustainable growth in broad leaf (dicot) weeds – corn, rice and wheat all have one leaf (monocot). Can be used for trees to hold fruit for development 2-4- D Cytokinins – Promote cell division and are produced in young leaves fruits and seeds. Used to stimulate cell division, induce shoot formation and auxiliary shoot proliferation while inhibiting root formation. Not good for stage III. Delays cell aging and increases as some fruits bloom and grow Used to induce bud growth in orchids and daylilies. Prevents browning in salads When mixed with gibberellins – can increase the size of a fruit (30-50% in pears and mangos) Ratio of Auxin and cytokinin control root formation Root initiation occurs when more auxin than cytokinin is in media and adventitious and shoot growth takes place when more cytokinin than auxin ratio. Gibberellins & Abciscic acid- Regulate cell elongation, determine plant height. Gibberellins increase growth of low-density cultures, enhance callus growth and elongate dwarf plants. Abscisic acid alters callus growth, enhance bud and shoot formation, and inhibit cell division. Commonly used in somatic embryogenesis – Ethylene- volatile gas produced during ripening, stress, mechanical damage or infection. Produced from methyl group of methionine Nearly all plant tissues can produce Natural role is to encourage fruit ripening and flower blooming Used commercially to initiate flowering and ripen tomatoes, citrus and bananas Specific protein receptors for ethylene have been found which act as transcription factors Can be a problem in culture without proper air circulation Design of Experiments Plackett-Burman Box-Behnken I. Fractional Factorial Designs As k increases, the runs specified for a 2k or 3k full factorial quickly become very large and outgrow the resources of most experimenters Solution: to assume that certain high-order interactions are negligible by using only a fraction of the runs specified by the full factorial design Properly chosen fractional factorial designs for two-level experiments have the desirable properties: – Balanced: All runs have the same number of observations) – Orthogonal: Effects of any factor sum to zero across the effects of the other factors) –II-Plackett-Burman –Plackett and Burman (1946) showed how full factorial designs can be fractionalized in a different manner than traditional 2k-p fractional factorial designs, in order to screen the max number of (main) effects in the least number of experimental runs. A. Properties & Assumptions : Fractional factorial designs for studying k = N – 1 variables in N runs, where N is a multiple of 4. Only main effects are of interest. Standard orthogonal arrays. No defining relation since interactions are not identically equal to main effects. All information is used to estimate the parameters leaving no degrees of freedom to estimate the error term for the ANOVA. B. When to use PB designs: Screening Possible to neglect higher order interactions 2-level multi-factor experiments. More than 4 factors, since for 2 to 4 variables a full factorial can be performed. To economically detect large main effects. Particularly useful for N = 12, 20, 24, 28 and 36. Experiment to study the eye focus time Response: Eye Focus time 1. Sharpness of vision 2. Distance from target to eye 3. Target shape 4. Illumination level 5. Target size 6. Target density 7. Subject. Screening experiment. Two levels of each factors are considered while higher order interactions are negligible Required to economically identify the most important factors. III. Box-Behnken Box-Behnken designs are the equivalent of Plackett-Burman designs for the case of 3**(k-p). A. Properties & Assumptions: Three-level multi-factor experiments. Combine two-level factorial designs with incomplete block designs. Complex confounding of interaction. Economical Fill out a polyhedron approximating a sphere. Can test the linear and quadratic (non-linear) effect for each factor. Standard orthogonal arrays. The treatment combinations are at the midpoints of edges of the process space and at the center. Rotatable Number of runs required by Central Composite and Box-Behnken designs Number of Box Behnken Central Composite factors 2 - 13 (5 center points) 3 15 20 (6 center point runs) 4 27 30 (6 center point runs) 5 46 33 (fractional factorial) or 52 (full factorial) 6 54 54 (fractional factorial) or 91 (full factorial) [source: Engineering Statistics Handbook] III. Box-Behnken B. When to use Box-Behnken designs: With three-level multi-factor experiments. When it is required to economically detect large main effects, especially when it is expensive to perform all the necessary runs. When it is required to determine the linear and quadratic effects of each variable. When the experimenter should avoid combined factor extremes. This property prevents a potential loss of data in those cases. III. Box-Behnken C. Example: Experiment to study the yield of a chemical process Response: Chemical process yield Factors: 1. Temperature 2. pH 3. Pressure 4. Viscosity 5. Mixer speed Screening experiment Three levels of each factors are considered while higher order interactions are negligible Required to economically identify the most important factors Summary of desirable features of Box-Behnken designs Satisfactory distribution of information across the experimental region (rotatability) Fitted values are as close as possible to observed values (minimize residuals or error of prediction) Good lack of fit detection. Internal estimate of error. Constant variance check. Transformations can be estimated. Suitability for blocking. Sequential construction of higher order designs from simpler designs Minimum number of treatment combinations. Good graphical analysis through simple data patterns. Good behavior when errors in settings of input variables occur. Response Surface Methods and Designs - Process Optimization These designs are useful for factor screening (i.e., identifying important factors that affect the performance of a process) Once the appropriate process variables have been identified, the next step is usually process optimization. Process optimization is the procedure of finding the set of operating conditions for the process variables that result in the best process performance. Response surface methodology is an approach to optimization developed in the early 1950s. Factorial Designs – 2 Level Design, with or without center point – Plackett – Burman Response Surface – Central Composite – Box-Behnken – 3 Level Factorial These methods is to find the best combination of factors values in order to optimize the system responses. Response surface methodology (RSM) is a collection of mathematical and statistical techniques that are useful for modeling and analysis in applications where a response is influenced by several variables. The objective of such an application is to optimize the response. In most RSM problems, the form of the relationship between the response and the independent variables is unknown. The first step in RSM is to find an approximation for the true relationship between the response, y, and the independent variables. Response surface methodology (RSM) uses various statistical, graphical, and mathematical techniques to develop, improve, or optimize a process. The most frequent applications of RSM are in the industrial area, where several predictor variables are used to predict some performance measure or quality characteristic of a product or process. RSM is a sequential procedure. The eventual objective of RSM is – to determine the optimum operating conditions for the system or – Determine a region of the factor space in which operating Sequential Nature of RSM ❖ We used designed experiments to determine what factors are important in determining the qualities of our product or the results of your process. ❖ Now we want to find factor settings or a factor region that optimize our response or responses. New Purpose Requires New Designs To estimate – linear parameters, we could use a two-level fractional factorial design of resolution ≥ 3. – cross-product parameters, we could use a fractional factorial design of resolution ≥ 5. – quadratic parameters, data for at least 3 levels of each factor is required. The Number of Levels Model adequacy requires testing (m+1) levels of a factor with order m polynomial effects. – Linear effects, m = 1, require two factor levels. – Quadratic effects, m = 2, require three factor levels. – Effects greater than second-order, m ≥ 3, are unusual. – We can avoid a large number of levels by restricting the factor ranges. Full factorial three level 3k K means number of factors and 3 is the number of levels The number of experiment is 3k RSM AS A SEQUENTIAL PROCESS The text has a graphic depicting a response surface method in three dimensions, though actually it is four dimensional space that is being represented , since the three factors are in 3-dimensional space , the response is the 4th dimension. Generation of matrix Model Analysis – ANOVA – Diagnostics – Graphical interpretation Optimization – Optimal conditions – Prediction of optimal conditions Uses of RSM To determine the factor levels that will simultaneously satisfy a set of desired specifications (e.g. model calibration). To determine the optimum combination of factors that yield the desired response and describe the response near the optimum To determine how a specific response is affected by changes in the level of the factors over the specified levels of interest. To achieve a quantitative understanding of the system behavior over the region tested To find conditions for process stability = insensitive spot (robust condition) To replace a more complex model with a much simpler second-order regression model for use within a limited range replacement models, meta models, or surrogate models. Design matrix (‘Design-Expert version 7·0’) The matrix F obtained from the factorial experiment. The factor levels are scaled so that its entries are coded as +1 and −1. A matrix E from the axial points, with 2k rows. Each factor is sequentially placed at ±α and all other factors are at zero. The value of α is determined by the designer; while arbitrary, some values may give the design desirable properties. Optimization scheme Two very useful and popular experimental designs that allow a 2nd order model to be fit are the: Central Composite Design (CCD) Box-Behnken Design (BBD) Both designs are built up from simple factorial or fractional factorial designs. Central Composite Design In statistics, a central composite design is an experimental design, useful in RSM, for building a second order (quadratic) model for the response variable without needing to use a complete three-level factorial experiment. A Box-Wilson Central Composite Design, commonly called 'a central composite design’. After the designed experiment is performed, linear regression is used, sometimes iteratively, to obtain results. Coded variables are often used when constructing this design. The design consists of three distinct sets of experimental runs: A factorial (perhaps fractional) design in the factors studied, each having two levels; A set of center points, experimental runs whose values of each factor are the medians of the values used in the factorial portion. This point is often replicated in order to improve the precision of the experiment; A set of axial points, experimental runs identical to the centre points except for one factor, which will take on values both below and above the median of the two factorial levels, and typically both outside their range. All factors are varied in this way. Summary of desirable features of Box-Behnken designs Satisfactory distribution of information across the experimental region (rotatability) Fitted values are as close as possible to observed values (minimize residuals or error of prediction) Good lack of fit detection. Internal estimate of error. Constant variance check. Transformations can be estimated. Suitability for blocking. Sequential construction of higher order designs from simpler designs Minimum number of treatment combinations. Good graphical analysis through simple data patterns. Good behavior when errors in settings of input variables occur. Kinetics of thermal death of microorganisms Steam (or moist heat) is used almost universally for the sterilization of fermentation media. Except the use of filtration for the sterilization of media for animal-cell culture – because such media are completely soluble and contain heat labile components making filtration is the method of choice for sterilization. The destruction of microorganism by heat is considered as loss of viability not destruction. The destruction of micro-organisms by steam (moist heat) at specific temperature can be described as a first-order chemical reaction provided if we considerer loss of viability not destruction. The thermal death kinetics may be represented by the following equation: -dN/dt = kd N 1 Where, – N, is the number of viable organisms present, – T, is the time of the sterilization treatment – kd, is the reaction rate constant of the reaction, or the specific death rate per time. On integration of equation (i) from t=0’ to t=t, we have the following expression : Nt/N0 =e-kdt 2 where – No is the number of viable organisms present at the start of the sterilization treatment, – Nt is the number of viable organisms present after a treatment period, t. On taking natural logarithms, equation (2) is reduced to: ln(Nt/N0) = - kd t 3 The graphically equations (1) and (3) are represented as, Plots of the proportion of survivors and the natural logarithm of the proportion of survivors in a population of microorganisms subjected to a lethal temperature over a time period. The relationship observed in the above graph would be found only with the sterilization of a pure culture in one physiological form, under ideal sterilization conditions. The term decimal reduction time, D, is used to characterize the death rate constant. D is defined as the sterilization time required to reduce the original number of viable cellsby one-tenth. N/N0 = 1/10 = e-kD ln(0.10) = -kD D = 2.303/k…………………………….(4) The thermal death rate constant k is given by Eq. 5 and follows the typical Arrhenius equation. K = A e-E/RT………..………………(5) Where: A = empirical constant E = Activation energy for thermal death of microorganism T = Absolute temperature, oK R = Gas constant = 1.98 cal/g mole oK In order to find the value of k for any system (spores and vegetative cells, nutrients) it is important to know both A and E in the Arrhenius Eq. 5. Sterilization at relatively high temperatures with short sterilization times is highly desirable because it favours the fast killing of vegetative cells and spores with minimal denaturation of nutrients present in the liquid medium. Types of equipment for batch sterilization of media Batch Sterilization Graph of generalized temperature-time profiles for the heating and cooling stages of a batch sterilization cycle Continuous Heat Sterilization of Liquids Continuous sterilization, particularly a high-temperature, short-exposure-time process, can reduce thermal damage to the medium significantly compared with batch sterilization, while achieving high levels of cell destruction. Other advantages include improved steam economy and more reliable scale-up. The amount of steam needed for continuous sterilization is 20 to 25% of that used in batch processes; the time required is also significantly reduced because heating and cooling are virtually instantaneous. In Figure (a), the raw medium entering the system is first preheated by the hot, sterile medium in a heat exchanger; this reduces the subsequent steam requirements for heating and cools the sterile medium. Steam is then injected directly into the medium as it flows through a pipe; as a result, the temperature of the medium rises almost instantaneously to the desired sterilization temperature. The time of exposure to this temperature depends on the length of pipe in the holding section of the sterilizer. After sterilization, the medium is cooled instantly by flash cooling, which is achieved by passing the liquid through an expansion valve into a vacuum chamber. Further cooling takes place in the heat exchanger where residual heat is used to preheat incoming medium. The sterile medium is then ready for use in the fermenter Continuous sterilizing equipment: (a) continuous steam injection with flash cooling; (b) heat transfer using heat exchangers Figure (b) shows an alternative sterilisation scheme based on heat exchange between the medium and steam. Raw medium is preheated using hot, sterile medium in a heat exchanger and is brought to the sterilisation temperature by further heat exchange with steam. The sterilisation temperature is maintained in the holding section; the sterile medium is then cooled by heat exchange with incoming medium before being used in the fermenter. Heat exchange systems are more expensive to construct than injection devices; fouling of the internal heat exchange surfaces also reduces the efficiency of heat transfer between cleanings. On the other hand, a disadvantage associated with steam injection is dilution of the medium by condensate; foaming from direct steam injection can also cause problems with operation of the flash cooler. The rates of heating and cooling in continuous sterilisers are much more rapid than in batch systems. Accordingly, in the design of continuous sterilisers, contributions to cell death outside of the holding period are generally ignored. An important variable affecting the performance of continuous sterilisers is the nature of the fluid flow in the system. Ideally, all fluid entering the equipment at a particular instant should spend the same time in the steriliser and exit the system at the same time. Plate heat exchanger type of continuous sterilization of liquid media. Variation of temperature with time in the continuous sterilizers of (a) continuous steam injection with flash cooling; (b) heat transfer using heat exchangers FILTER STERILISATION OF LIQUIDS Sometimes, fermentation media or selected medium ingredients are sterilised by filtration rather than by heat. Medium containing heat-labile components such as serum, differentiation factors, enzymes, or other proteins is easily destroyed by heat and must be sterilised by other means. Typically, the membranes used for filter sterilisation of liquids are made of cellulose esters or other polymers and have pore diameters between 0.2 and 0.45 μm. As medium passes through the filter, bacteria and other particles with dimensions greater than the pore size are screened out and collect on the surface of the membrane. The small pore sizes used in liquid filtration mean that the membranes readily become blocked unless the medium is prefiltered to remove any large particles. To achieve high filtration flow rates, large membrane surface areas are required. The membranes themselves must be sterilised by steam or radiation before use; alternatively, disposable filters and filter cartridges are used. Filtration using pore sizes of 0.2 to 0.45 μm may not be as effective or reliable as heat sterilisation because viruses and mycoplasma are able to pass through the membranes. However, the availability of 0.1-μm sterilising-grade membrane filters for mycoplasma removal has seen the widespread adoption of filter sterilisation in many mammalian cell culture processes where complex, proteinaceous media are used routinely STERILISATION OF AIR In aerobic fermentation systems sterile air must be provided: To the bioreactor vessel as the source of oxygen for the metabolic activity and growth of the microorganisms. For the aseptic operation of the bioreactor system. The ambient air contains: Dust and other inert particles Bacteria, spores, and other undesirable contaminant microorganisms. The number of microbial cells in the air is of the order 103 to 104 m3. Filtration is the most common method used to sterilize air in large-scale bioprocesses; heat sterilization of gases is economically impractical. Depth filters consisting of compacted beds or pads of fibrous material such as glass wool have been used widely in the fermentation industry. Distances between the fibres in depth filters are typically 2 to 10 μm, or up to 10 times greater than the dimensions of the bacteria and spores to be removed. Airborne particles penetrate the bed to various depths before their passage through the filter is arrested; the depth of the filter required to produce air of sufficient quality depends on the operating flow rate and the incoming level of contamination. Cells are collected in depth filters by a combination of impaction, interception, electrostatic effects, and, for particles smaller than about 1.0 μm, diffusion to the fibres. Depth filters do not perform well if there are large fluctuations in air flow rate or if the air is wet: liquid condensing in the filter increases the pressure drop, causes channeling of the gas flow, and provides a pathway for organisms collected on the fibres to grow through the bed. Increasingly, depth filters are being replaced in industrial applications by membrane cartridge filters. These filters use steam-sterilizable or disposable polymeric membranes that act as surface filters trapping contaminants as on a sieve. Working of Autoclave. High-efficiency particulate air (HEPA) filters are used to filter the air flowing into aseptic environments ( e.g. operating rooms) and out of potentially contaminated ones (e.g., containment facilities) Most membranes are steam sterilizable. Membrane filter cartridges typically contain a pleated, hydrophobic filter with small and uniformly sized pores of diameter 0.45 μm or less. The hydrophobic nature of the surface minimises problems with filter wetting while the pleated configuration allows a high filtration area to be packed into a small cartridge volume. Membrane air filters are usually made of the following materials: Ceramic materials: Durable, can be backwashed, steam sterilizable, can be used many times, economical Many other polymeric materials, such as: polyvinyl alcohol (PVA), cellulose acetate, Polysulfone, Other composite polymeric materials A pre-filter is used before the main filtration membrane to remove large size particles and other contaminants. This protects the main membranes from plugging. Prefilters built into the cartridge or located upstream reduce fouling of the membrane by removing large particles, oil, water droplets, and foam from the incoming gas. Filters are also used to sterilise off-gases leaving fermenters. In this case, the objective is to prevent release into the atmosphere of any organisms entrained in aerosols in the headspace of the reactor. The concentration of cells in unfiltered fermenter off-gas is several times greater than in air. Containment is particularly important when the organisms used in fermentation processes are potentially harmful to plant personnel or the environment. Companies operating fermentations with pathogenic or recombinant strains are required by regulatory authorities to prevent the cells from escaping into the atmosphere Filtration mechanism Inertial impaction Interception Diffusion Electrostatic force Settling due to gravitation Kinetics: dN/dt = -KN ----------- (1) N – Number of particles at a depth of ‘X’ in the filter K – Constant Integrating (1), No/Nt = e –kx ------ (2) No – Number of particles at the entrance of filter bed at X =0 From (2), ln (Nt/ No) = -KX --------- (3) Efficiency – Ratio of number of particles removed to the initial number of particles, Ef = No-Nt/No = 1-Nt/No Ef = 1- e-KX X90 - Depth of filter required to remove 90% of the solids entering the filter, X90 = 2.303/K ---- Proposed by Humphery and Gaden K – function of filter medium and air velocity.