Physiology (6th Edition) PDF
Document Details
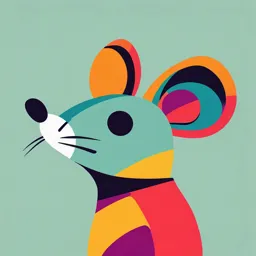
Uploaded by EventfulNihonium
Medical College of Virginia, Virginia Commonwealth University
2015
Linda S. Costanzo
Tags
Related
Summary
This book, "Physiology (6th Edition)", is a review of key physiological principles designed to assist students preparing for the USMLE Step 1 exam. Organized by organ system, it covers cellular, neuro, cardiovascular, respiratory, renal and acid-base, gastrointestinal, and endocrine physiology. Detailed explanations and illustrations aid in understanding.
Full Transcript
Physiology S i x t h E d i t i o n 0002069208.INDD 1 2/13/2014 2:34:54 PM 0002069208.INDD 2 2/13/2014 2:34:55 PM iii Physiology S i...
Physiology S i x t h E d i t i o n 0002069208.INDD 1 2/13/2014 2:34:54 PM 0002069208.INDD 2 2/13/2014 2:34:55 PM iii Physiology S i x t h E d i t i o n Linda S. Costanzo, Ph.D. Professor of Physiology and Biophysics Medical College of Virginia Virginia Commonwealth University Richmond, Virginia 0002069208.INDD 3 2/13/2014 2:34:56 PM Publisher: Michael Tully Acquisitions Editor: Crystal Taylor Product Development Editors: Stacey Sebring and Amy Weintraub Production Project Manager: David Saltzberg Marketing Manager: Joy Fisher-Williams Designer: Holly Reid McLaughlin Manufacturing Coordinator: Margie Orzech Compositor: SPi Global 6th Edition Copyright © 2015, 2011, 2007, 2003, 1998, 1995 Wolters Kluwer Health. 351 West Camden Street Two Commerce Square Baltimore, MD 21201 2001 Market Street Philadelphia, PA 19103 Printed in China All rights reserved. This book is protected by copyright. No part of this book may be reproduced or transmitted in any form or by any means, including as photocopies or scanned-in or other electronic copies, or utilized by any information storage and retrieval system without written permission from the copyright owner, except for brief quotations embodied in critical articles and reviews. Materials appearing in this book prepared by individuals as part of their official duties as US government employees are not covered by the above-mentioned copyright. To request permission, please contact Lippincott Williams & Wilkins at 2001 Market Street, Philadelphia, PA 19103, via email at [email protected], or via website at lww.com (products and services). 9 8 7 6 5 4 3 2 1 Library of Congress Cataloging-in-Publication Data Costanzo, Linda S., 1947- author. Physiology / Linda S. Costanzo. — Sixth edition. p. ; cm. — (Board review series) Includes index. ISBN 978-1-4511-8795-3 I. Title. II. Series: Board review series. [DNLM: 1. Physiological Phenomena—Examination Questions. 2. Physiology—Examination Questions. QT 18.2] QP40 612'.0076—dc23 2013045098 DISCLAIMER Care has been taken to confirm the accuracy of the information present and to describe generally accepted practices. However, the authors, editors, and publisher are not responsible for errors or omissions or for any consequences from application of the information in this book and make no warranty, expressed or implied, with respect to the currency, completeness, or accuracy of the contents of the publication. Application of this information in a particular situation remains the professional responsibility of the practitioner; the clinical treatments described and recommended may not be considered absolute and universal recommendations. The authors, editors, and publisher have exerted every effort to ensure that drug selection and dosage set forth in this text are in accordance with the current recommendations and practice at the time of publication. However, in view of ongoing research, changes in government regulations, and the constant flow of information relating to drug therapy and drug reactions, the reader is urged to check the package insert for each drug for any change in indications and dosage and for added warnings and precautions. This is particularly important when the recommended agent is a new or infrequently employed drug. Some drugs and medical devices presented in this publication have Food and Drug Administration (FDA) clearance for limited use in restricted research settings. It is the responsibility of the health care provider to ascertain the FDA status of each drug or device planned for use in their clinical practice. To purchase additional copies of this book, call our customer service department at (800) 638-3030 or fax orders to (301) 223-2320. International customers should call (301) 223-2300. Visit Lippincott Williams & Wilkins on the Internet: http://www.lww.com. Lippincott Williams & Wilkins customer service representatives are available from 8:30 am to 6:00 pm, EST. 0002069208.INDD 4 2/13/2014 2:34:56 PM For Richard And for Dan, Rebecca, and Sheila And for Elise and Max 0002069208.INDD 5 2/13/2014 2:34:56 PM Preface The subject matter of physiology is the foundation of the practice of medicine, and a firm grasp of its principles is essential for the physician. This book is intended to aid the student prepar- ing for the United States Medical Licensing Examination (USMLE) Step 1. It is a concise review of key physiologic principles and is intended to help the student recall material taught during the first and second years of medical school. It is not intended to substitute for comprehensive textbooks or for course syllabi, although the student may find it a useful adjunct to physiology and pathophysiology courses. The material is organized by organ system into seven chapters. The first chapter reviews general principles of cellular physiology. The remaining six chapters review the major organ systems—neurophysiology, cardiovascular, respiratory, renal and acid–base, gastrointestinal, and endocrine physiology. Difficult concepts are explained stepwise, concisely, and clearly, with appropriate illustra- tive examples and sample problems. Numerous clinical correlations are included so that the student can understand physiology in relation to medicine. An integrative approach is used, when possible, to demonstrate how the organ systems work together to maintain homeostasis. More than 130 full-color illustrations and flow diagrams and more than 50 tables help the stu- dent visualize the material quickly and aid in long-term retention. The inside front cover con- tains “Key Physiology Topics for USMLE Step 1.” The inside back cover contains “Key Physiology Equations for USMLE Step 1.” Questions reflecting the content and format of USMLE Step 1 are included at the end of each chapter and in a Comprehensive Examination at the end of the book. These questions, many with clinical relevance, require problem-solving skills rather than straight recall. Clear, concise explanations accompany the questions and guide the student through the correct steps of reasoning. The questions can be used as a pretest to identify areas of weakness or as a posttest to determine mastery. Special attention should be given to the Comprehensive Examination, because its questions integrate several areas of physiology and related concepts of pathophysi- ology and pharmacology. New to this edition: Addition of new full-color figures Updated organization and text Expanded coverage of cellular, respiratory, renal, gastrointestinal, and endocrine physiology Increased emphasis on pathophysiology Best of luck in your preparation for USMLE Step 1! Linda S. Costanzo, Ph.D. vi 0002069208.INDD 6 2/13/2014 2:34:56 PM Acknowledgments It has been a pleasure to be a part of the Board Review Series and to work with the staff at Lippincott Williams & Wilkins. Crystal Taylor and Stacey Sebring provided expert editorial assistance. My sincere thanks to students in the School of Medicine at Virginia Commonwealth University/Medical College of Virginia, who have provided so many helpful suggestions for BRS Physiology. Thanks also to the many students from other medical schools who have taken the time to write to me about their experiences with this book. Linda S. Costanzo, Ph.D. vii 0002069208.INDD 7 2/13/2014 2:34:56 PM Contents Preface vi Acknowledgments vii 1. CELL PHYSIOLOGY 1 I. Cell Membranes 1 II. Transport Across Cell Membranes 2 III. Osmosis 4 IV. Diffusion Potential, Resting Membrane Potential, and Action Potential 7 V. Neuromuscular and Synaptic Transmission 12 VI. Skeletal Muscle 16 VII. Smooth Muscle 20 VIII. Comparison of Skeletal Muscle, Smooth Muscle, and Cardiac Muscle 22 Review Test 23 2. NEUROPHYSIOLOGY 32 I. Autonomic Nervous System (ANS) 32 II. Sensory Systems 36 III. Motor Systems 48 IV. Higher Functions of the Cerebral Cortex 54 V. Blood–Brain Barrier and Cerebrospinal Fluid (CSF) 55 VI. Temperature Regulation 56 Review Test 58 3. CARDIOVASCULAR PHYSIOLOGY 66 I. Circuitry of the Cardiovascular System 66 II. Hemodynamics 66 III. Cardiac Electrophysiology 71 IV. Cardiac Muscle and Cardiac Output 76 V. Cardiac Cycle 85 viii 0002069208.INDD 8 2/13/2014 2:34:57 PM Contents ix VI. Regulation of Arterial Pressure 87 VII. Microcirculation and Lymph 91 VIII. Special Circulations 94 IX. Integrative Functions of the Cardiovascular System: Gravity, Exercise, and Hemorrhage 97 Review Test 102 4. RESPIRATORY PHYSIOLOGY 115 I. Lung Volumes and Capacities 115 II. Mechanics of Breathing 117 III. Gas Exchange 124 IV. Oxygen Transport 126 V. CO2 Transport 131 VI. Pulmonary Circulation 132 VII. V/Q Defects 133 VIII. Control of Breathing 135 IX. Integrated Responses of the Respiratory System 137 Review Test 139 5. RENAL AND ACID–BASE PHYSIOLOGY 147 I. Body Fluids 147 II. Renal Clearance, Renal Blood Flow (RBF), and Glomerular Filtration Rate (GFR) 151 III. Reabsorption and Secretion 155 IV. NaCl Regulation 158 V. K+ Regulation 163 VI. Renal Regulation of Urea, Phosphate, Calcium, and Magnesium 166 VII. Concentration and Dilution of Urine 167 VIII. Renal Hormones 172 IX. Acid–Base Balance 172 X. Diuretics 181 XI. Integrative Examples 181 Review Test 184 6. GASTROINTESTINAL PHYSIOLOGY 194 I. Structure and Innervation of the Gastrointestinal Tract 194 II. Regulatory Substances in the Gastrointestinal Tract 195 III. Gastrointestinal Motility 199 IV. Gastrointestinal Secretion 204 V. Digestion and Absorption 214 VI. Liver Physiology 219 Review Test 221 0002069208.INDD 9 2/13/2014 2:34:57 PM x Contents 7. ENDOCRINE PHYSIOLOGY 227 I. Overview of Hormones 227 II. Cell Mechanisms and Second Messengers 229 III. Pituitary Gland (Hypophysis) 233 IV. Thyroid Gland 238 V. Adrenal Cortex and Adrenal Medulla 241 VI. Endocrine Pancreas–Glucagon and Insulin 248 VII. Calcium Metabolism (Parathyroid Hormone, Vitamin D, Calcitonin) 251 VIII. Sexual Differentiation 255 IX. Male Reproduction 256 X. Female Reproduction 258 Review Test 263 Comprehensive Examination 271 Index 293 0002069208.INDD 10 2/13/2014 2:34:57 PM chapter 1 Cell Physiology I. Cell Membranes are composed primarily of phospholipids and proteins. A. Lipid bilayer 1. Phospholipids have a glycerol backbone, which is the hydrophilic (water soluble) head, and two fatty acid tails, which are hydrophobic (water insoluble). The hydrophobic tails face each other and form a bilayer. 2. Lipid-soluble substances (e.g., O2, CO2, steroid hormones) cross cell membranes because they can dissolve in the hydrophobic lipid bilayer. 3. Water-soluble substances (e.g., Na+, Cl−, glucose, H2O) cannot dissolve in the lipid of the membrane, but may cross through water-filled channels, or pores, or may be trans- ported by carriers. B. Proteins 1. Integral proteins are anchored to, and imbedded in, the cell membrane through hydrophobic interactions. may span the cell membrane. include ion channels, transport proteins, receptors, and guanosine 5′-triphosphate (GTP)–binding proteins (G proteins). 2. Peripheral proteins are not imbedded in the cell membrane. are not covalently bound to membrane components. are loosely attached to the cell membrane by electrostatic interactions. C. Intercellular connections 1. Tight junctions (zonula occludens) are the attachments between cells (often epithelial cells). may be an intercellular pathway for solutes, depending on the size, charge, and charac- teristics of the tight junction. may be “tight” (impermeable), as in the renal distal tubule, or “leaky” (permeable), as in the renal proximal tubule and gallbladder. 2. Gap junctions are the attachments between cells that permit intercellular communication. for example, permit current flow and electrical coupling between myocardial cells. 1 0002069201.INDD 1 2/12/2014 10:45:54 AM 2 BRS Physiology t a b l e 1.1 Characteristics of Different Types of Transport Electrochemical Carrier- Metabolic Na+ Inhibition of Type Gradient Mediated Energy Gradient Na+–K+ Pump Simple diffusion Downhill No No No — Facilitated diffusion Downhill Yes No No — Primary active Uphill Yes Yes — Inhibits (if transport Na+–K+ pump) Cotransport Uphill* Yes Indirect Yes, same Inhibits direction Countertransport Uphill* Yes Indirect Yes, Inhibits opposite direction * One or more solutes are transported uphill; Na+ is transported downhill. II. Transport Across Cell Membranes (Table 1.1) A. Simple diffusion 1. Characteristics of simple diffusion is the only form of transport that is not carrier mediated. occurs down an electrochemical gradient (“downhill”). does not require metabolic energy and therefore is passive. 2. Diffusion can be measured using the following equation: J = −PA (C1 − C2 ) where: J = flux (flow) (mmol/sec) P = permeability (cm/sec) A = area (cm2) C1 = concentration1 (mmol/L) C2 = concentration2 (mmol/L) 3. Sample calculation for diffusion Theurea concentration of blood is 10 mg/100 mL. The urea concentration of proximal tubular fluid is 20 mg/100 mL. If the permeability to urea is 1 × 10−5 cm/sec and the sur- face area is 100 cm2, what are the magnitude and direction of the urea flux? 1 × 10−5 cm 2 20 mg 10 mg Flux = sec ( ) 100 cm 100 mL − 100 mL 1 × 10−5 cm 10 mg = sec ( ) 100 cm 2 100 mL 1 × 10 cm −5 2 0.1 mg = sec ( ) 100 cm cm 3 = 1 × 10−4 mg /sec from lumen to blood ( high to low concentration ) Note: The minus sign preceding the diffusion equation indicates that the direction of flux, or flow, is from high to low concentration. It can be ignored if the higher concen- tration is called C1 and the lower concentration is called C2. Also note: 1 mL = 1 cm3. 0002069201.INDD 2 2/12/2014 10:45:55 AM Chapter 1 Cell Physiology 3 4. Permeability is the P in the equation for diffusion. describes the ease with which a solute diffuses through a membrane. depends on the characteristics of the solute and the membrane. a. F actors that increase permeability: ↑ Oil/water partition coefficient of the solute increases solubility in the lipid of the membrane. ↓ Radius (size) of the solute increases the diffusion coefficient and speed of diffusion. ↓ Membrane thickness decreases the diffusion distance. b. Small hydrophobic solutes (e.g., O2, CO2) have the highest permeabilities in lipid membranes. c. Hydrophilic solutes (e.g., Na+, K+) must cross cell membranes through water-filled channels, or pores, or via transporters. If the solute is an ion (is charged), then its flux will depend on both the concentration difference and the potential difference across the membrane. B. Carrier-mediated transport includes facilitated diffusion and primary and secondary active transport. The characteristics of carrier-mediated transport are 1. Stereospecificity. For example, d-glucose (the natural isomer) is transported by facilitated diffusion, but the l-isomer is not. Simple diffusion, in contrast, would not distinguish between the two isomers because it does not involve a carrier. 2. Saturation. The transport rate increases as the concentration of the solute increases, until the carriers are saturated. The transport maximum (Tm) is analogous to the maximum velocity (Vmax) in enzyme kinetics. 3. Competition. Structurally related solutes compete for transport sites on carrier m olecules. For example, galactose is a competitive inhibitor of glucose transport in the small intestine. C. Facilitated diffusion 1. Characteristics of facilitated diffusion occurs down an electrochemical gradient (“downhill”), similar to simple diffusion. does not require metabolic energy and therefore is passive. is more rapid than simple diffusion. is carrier mediated and therefore exhibits stereospecificity, saturation, and competition. 2. Example of facilitated diffusion Glucose transport in muscle and adipose cells is “downhill,” is carrier-mediated, and is inhibited by sugars such as galactose; therefore, it is categorized as facilitated diffusion. In diabetes mellitus, glucose uptake by muscle and adipose cells is impaired because the carriers for facilitated diffusion of glucose require insulin. D. Primary active transport 1. Characteristics of primary active transport occurs against an electrochemical gradient (“uphill”). requires direct input of metabolic energy in the form of adenosine triphosphate (ATP) and therefore is active. is carrier mediated and therefore exhibits stereospecificity, saturation, and competition. 2. Examples of primary active transport a. Na+, K+-ATPase (or Na+–K+ pump) in cell membranes transports Na+ from intracellular to extracellular fluid and K+ from extracellular to intracellular fluid; it maintains low intracellular [Na+] and high intracellular [K+]. 0002069201.INDD 3 2/12/2014 10:45:55 AM 4 BRS Physiology + Both Na and K+ are transported against their electrochemical gradients. Energy is provided from the terminal phosphate bond of ATP. The usual stoichiometry is 3 Na+/2 K+. Specific inhibitors of Na+, K+-ATPase are the cardiac glycoside drugs ouabain and digitalis. b. Ca2+-ATPase (or Ca2+ pump) in the sarcoplasmic reticulum (SR) or cell membranes trans- ports Ca2+ against an electrochemical gradient. Sarcoplasmic and endoplasmic reticulum Ca -ATPase is called SERCA. 2+ + + c. H , K -ATPase (or proton pump) in gastric parietal cells transports H+ into the lumen of the stomach against its electrochemical gradient. It is inhibited by proton pump inhibitors, such as omeprazole. E. Secondary active transport 1. Characteristics of secondary active transport a. The transport of two or more solutes is coupled. b. One of the solutes (usually Na+) is transported “downhill” and provides energy for the “uphill” transport of the other solute(s). c. Metabolic energy is not provided directly but indirectly from the Na+ gradient that is maintained across cell membranes. Thus, inhibition of Na+, K+-ATPase will decrease transport of Na+ out of the cell, decrease the transmembrane Na+ gradient, and eventu- ally inhibit secondary active transport. d. If the solutes move in the same direction across the cell membrane, it is called c otransport or symport. + Examples are Na -glucose cotransport in the small intestine and renal early proximal tubule and Na –K+–2Cl– cotransport in the renal thick ascending limb. + e. If the solutes move in opposite directions across the cell membranes, it is called countertransport, exchange, or antiport. + 2+ + + Examples are Na -Ca exchange and Na –H exchange. + 2. Example of Na –glucose cotransport (Figure 1.1) a. The carrier for Na+–glucose cotransport is located in the luminal membrane of intesti- nal mucosal and renal proximal tubule cells. b. G lucose is transported “uphill”; Na+ is transported “downhill.” c. Energy is derived from the “downhill” movement of Na+. The inwardly directed Na+ gradient is maintained by the Na+–K+ pump on the basolateral (blood side) membrane. Poisoning the Na+–K+ pump decreases the transmembrane Na+ gradient and conse- quently inhibits Na+–glucose cotransport. 3. Example of Na+–Ca2+ countertransport or exchange (Figure 1.2) a. Many cell membranes contain a Na+–Ca2+ exchanger that transports Ca2+ “uphill” from low intracellular [Ca2+] to high extracellular [Ca2]. Ca2+ and Na+ move in opposite direc- tions across the cell membrane. b. The energy is derived from the “downhill” movement of Na+. As with cotransport, the inwardly directed Na+ gradient is maintained by the Na+–K+ pump. Poisoning the Na+– K+ pump therefore inhibits Na+–Ca2+ exchange. III. Osmosis A. Osmolarity is the concentration of osmotically active particles in a solution. is a colligative property that can be measured by freezing point depression. 0002069201.INDD 4 2/12/2014 10:45:56 AM Chapter 1 Cell Physiology 5 Lumen Intestinal or Blood proximal tubule cell Na+ Na+ Na+ Na+ Glucose K+ Na+ + Secondary Primary Figure 1.1 Na –glucose cotransport (symport) in intestinal or proximal tubule epithelial cell. active active can be calculated using the following equation: Osmolarity = g ¥ C where: Osmolarity = concentration of particles (Osm/L) g = number of particles in solution (Osm/mol) [e.g., gNaCl = 2; gglucose = 1] C = concentration (mol/L) Two solutions that have the same calculated osmolarity are isosmotic. If two solutions have different calculated osmolarities, the solution with the higher osmolarity is hyperosmotic and the solution with the lower osmolarity is hyposmotic. Sample calculation: What is the osmolarity of a 1 M NaCl solution? Osmolarity = g × C = 2 Osm/mol × 1M = 2 Osm/L B. Osmosis and osmotic pressure Osmosisis the flow of water across a semipermeable membrane from a solution with low solute concentration to a solution with high solute concentration. 1. Example of osmosis (Figure 1.3) a. Solutions 1 and 2 are separated by a semipermeable membrane. Solution 1 contains a solute that is too large to cross the membrane. Solution 2 is pure water. The presence of the solute in solution 1 produces an osmotic pressure. b. The osmotic pressure difference across the membrane causes water to flow from solu- tion 2 (which has no solute and the lower osmotic pressure) to solution 1 (which has the solute and the higher osmotic pressure). c. With time, the volume of solution 1 increases and the volume of solution 2 decreases. Secondary active Na+ Ca2+ Ca2+ Ca2+ Na+ Na+ Na+ K+ Primary + 2+ Figure 1.2 Na –Ca countertransport (antiport). active 0002069201.INDD 5 2/12/2014 10:45:57 AM 6 BRS Physiology Semipermeable membrane Time Water flows by osmosis from 2 1 1 2 1 2 Figure 1.3 Osmosis of H2O across a semipermeable membrane. alculating osmotic pressure (van’t Hoff’s law) 2. C a. The osmotic pressure of solution 1 (see Figure 1.3) can be calculated by van’t Hoff’s law, which states that osmotic pressure depends on the concentration of osmotically active particles. The concentration of particles is converted to pressure according to the fol- lowing equation: p = g ¥ C ¥ RT where: π = osmotic pressure (mm Hg or atm) g = number of particles in solution (osm/mol) C = concentration (mol/L) R = gas constant (0.082 L—atm/mol—K) T = absolute temperature (K) b. The osmotic pressure increases when the solute concentration increases. A solution of 1 M CaCl2 has a higher osmotic pressure than a solution of 1 M KCl because the con- centration of particles is higher. c. T he higher the osmotic pressure of a solution, the greater the water flow into it. d. Two solutions having the same effective osmotic pressure are isotonic because no water flows across a semipermeable membrane separating them. If two solutions separated by a semipermeable membrane have different effective osmotic pressures, the solu- tion with the higher effective osmotic pressure is hypertonic and the solution with the lower effective osmotic pressure is hypotonic. Water flows from the hypotonic to the hypertonic solution. e. Colloid osmotic pressure, or oncotic pressure, is the osmotic pressure created by pro- teins (e.g., plasma proteins). 3. Reflection coefficient (σ) a number between zero and one that describes the ease with which a solute perme- is ates a membrane. a. If the reflection coefficient is one, the solute is impermeable. Therefore, it is retained in the original solution, it creates an osmotic pressure, and it causes water flow. Serum albumin (a large solute) has a reflection coefficient of nearly one. b. If the reflection coefficient is zero, the solute is completely permeable. Therefore, it will not exert any osmotic effect, and it will not cause water flow. Urea (a small solute) usually has a reflection coefficient of close to zero and it is, therefore, an ineffective osmole. 4. C alculating effective osmotic pressure Effective osmotic pressure is the osmotic pressure (calculated by van’t Hoff’s law) mul- tiplied by the reflection coefficient. If the reflection coefficient is one, the solute will exert maximal effective osmotic pres- sure. If the reflection coefficient is zero, the solute will exert no osmotic pressure. 0002069201.INDD 6 2/12/2014 10:46:00 AM Chapter 1 Cell Physiology 7 IV. D iffusion Potential, Resting Membrane Potential, and Action Potential A. Ion channels are integral proteins that span the membrane and, when open, permit the passage of cer- tain ions. 1. Ion channels are selective; they permit the passage of some ions, but not others. Selectivity is based on the size of the channel and the distribution of charges that line it. For example, a small channel lined with negatively charged groups will be selective for small cations and exclude large solutes and anions. Conversely, a small channel lined with positively charged groups will be selective for small anions and exclude large sol- utes and cations. 2. Ion channels may be open or closed. When the channel is open, the ion(s) for which it is selective can flow through. When the channel is closed, ions cannot flow through. 3. The conductance of a channel depends on the probability that the channel is open. The higher the probability that a channel is open, the higher the conductance, or permeabil- ity. Opening and closing of channels are controlled by gates. a. Voltage-gated channels are opened or closed by changes in membrane potential. + The activation gate of the Na channel in nerve is opened by depolarization; when open, the nerve membrane is permeable to Na+ (e.g., during the upstroke of the nerve action potential). + The inactivation gate of the Na channel in nerve is closed by depolarization; when closed, the nerve membrane is impermeable to Na+ (e.g., during the repolarization phase of the nerve action potential). b. Ligand-gated channels are opened or closed by hormones, second messengers, or neurotransmitters. example, the nicotinic receptor for acetylcholine (ACh) at the motor end plate is For an ion channel that opens when ACh binds to it. When open, it is permeable to Na+ and K+, causing the motor end plate to depolarize. B. Diffusion and equilibrium potentials A diffusion potential is the potential difference generated across a membrane because of a concentration difference of an ion. A diffusion potential can be generated only if the membrane is permeable to the ion. The size of the diffusion potential depends on the size of the concentration gradient. The sign of the diffusion potential depends on whether the diffusing ion is positively or negatively charged. Diffusion potentials are created by the diffusion of very few ions and, therefore, do not result in changes in concentration of the diffusing ions. The equilibrium potential is the potential difference that would exactly balance (oppose) the tendency for diffusion down a concentration difference. At electrochemical equil ibrium, the chemical and electrical driving forces that act on an ion are equal and opposite, and no more net diffusion of the ion occurs. 1. Example of a Na+ diffusion potential (Figure 1.4) a. Two solutions of NaCl are separated by a membrane that is permeable to Na+ but not to Cl−. The NaCl concentration of solution 1 is higher than that of solution 2. b. Because the membrane is permeable to Na+, Na+ will diffuse from solution 1 to solution 2 down its concentration gradient. Cl− is impermeable and therefore will not accom- pany Na+. c. As a result, a diffusion potential will develop and solution 1 will become negative with respect to solution 2. 0002069201.INDD 7 2/12/2014 10:46:01 AM 8 BRS Physiology Na+-selective membrane 1 2 1 2 Na+ Na+ Na+ – + Na+ – + – + Cl– Cl– – + Cl– Cl– Figure 1.4 Generation of an Na+ diffusion potential across a Na+-selective membrane. d. Eventually, the potential difference will become large enough to oppose further net diffusion of Na+. The potential difference that exactly counterbalances the diffusion of Na+ down its concentration gradient is the Na+ equilibrium potential. At electrochemical equilibrium, the chemical and electrical driving forces on Na+ are equal and opposite, and there is no net diffusion of Na+. 2. Example of a Cl− diffusion potential (Figure 1.5) a. Two solutions identical to those shown in Figure 1.4 are now separated by a membrane that is permeable to Cl− rather than to Na+. l− will diffuse from solution 1 to solution 2 down its concentration gradient. Na+ is b. C impermeable and therefore will not accompany Cl−. c. A diffusion potential will be established such that solution 1 will become positive with respect to solution 2. The potential difference that exactly counterbalances the diffu- sion of Cl− down its concentration gradient is the Cl− equilibrium potential. At electro- chemical equilibrium, the chemical and electrical driving forces on Cl− are equal and opposite, and there is no net diffusion of Cl−. 3. Using the Nernst equation to calculate equilibrium potentials a. T he Nernst equation is used to calculate the equilibrium potential at a given concentra- tion difference of a permeable ion across a cell membrane. It tells us what potential would exactly balance the tendency for diffusion down the concentration gradient; in other words, at what potential would the ion be at electrochemical equilibrium? E = -2.3 RT [C ] log10 i zF [C e ] where: E = equilibrium potential (mV) RT 60 mV 2.3 = at 37°C zF z z = charge on the ion (+1 for Na+, +2 for Ca2+, −1 for Cl−) Ci = intracellular concentration (mM) Ce = extracellular concentration (mM) Cl–-selective membrane 1 2 1 2 Na+ Na+ + – Na+ + – Na+ + – + – Cl– Cl– Cl– Cl– Figure 1.5 Generation of a Cl− diffusion potential across a Cl−-selective membrane. 0002069201.INDD 8 2/12/2014 10:46:04 AM Chapter 1 Cell Physiology 9 b. Sample calculation with the Nernst equation the intracellular [Na+] is 15 mM and the extracellular [Na+] is 150 mM, what is the If equilibrium potential for Na+? E Na + = −60 mV [C ] log10 i z [C e ] −60 mV 15 mM = log10 +1 150 mM = −60 mV log10 0.1 = +60 mV Note: You need not remember which concentration goes in the numerator. Because it is a log function, perform the calculation either way to get the absolute value of 60 mV. Then use an “intuitive approach” to determine the correct sign. (Intuitive approach: The [Na+] is higher in extracellular fluid than in intracellular fluid, so Na+ ions will diffuse from extracellular to intracellular, making the inside of the cell positive [i.e., +60 mV at equilibrium].) c. Approximate values for equilibrium potentials in nerve and muscle E Na + +65 mV ECa 2+ +120 mV EK+ −85 mV ECl − −85 mV C. Driving force and current flow The driving force on an ion is the difference between the actual membrane potential (Em) and the ion’s equilibrium potential (calculated with the Nernst equation). Current flow occurs if there is a driving force on the ion and the membrane is permeable to the ion. The direction of current flow is in the same direction as the driving force. The magnitude of current flow is determined by the size of the driving force and the perme- ability (or conductance) of the ion. If there is no driving force on the ion, no current flow can occur. If the membrane is impermeable to the ion, no current flow can occur. D. Resting membrane potential is expressed as the measured potential difference across the cell membrane in millivolts (mV). is, by convention, expressed as the intracellular potential relative to the extracellular potential. Thus, a resting membrane potential of −70 mV means 70 mV, cell negative. 1. The resting membrane potential is established by diffusion potentials that result from con- centration differences of permeant ions. 2. Each permeable ion attempts to drive the membrane potential toward its equilibrium poten- tial. Ions with the highest permeabilities, or conductances, will make the greatest contri- butions to the resting membrane potential, and those with the lowest permeabilities will make little or no contribution. 3. For example, the resting membrane potential of nerve is −70 mV, which is close to the cal- culated K+ equilibrium potential of −85 mV, but far from the calculated Na+ equilibrium potential of +65 mV. At rest, the nerve membrane is far more permeable to K+ than to Na+. 4. The Na+–K+ pump contributes only indirectly to the resting membrane potential by main- taining, across the cell membrane, the Na+ and K+ concentration gradients that then produce diffusion potentials. The direct electrogenic contribution of the pump (3 Na+ pumped out of the cell for every 2 K+ pumped into the cell) is small. 0002069201.INDD 9 2/12/2014 10:46:07 AM 10 BRS Physiology E. Action potentials 1. Definitions a. Depolarization makes the membrane potential less negative (the cell interior becomes less negative). b. Hyperpolarization makes the membrane potential more negative (the cell interior becomes more negative). c. Inward current is the flow of positive charge into the cell. Inward current depolarizes the membrane potential. d. Outward current is the flow of positive charge out of the cell. Outward current hyperpo- larizes the membrane potential. e. Action potential is a property of excitable cells (i.e., nerve, muscle) that consists of a rapid depolarization, or upstroke, followed by repolarization of the membrane potential. Action potentials have stereotypical size and shape, are propagating, and are all-or-none. f. Threshold is the membrane potential at which the action potential is inevitable. At threshold potential, net inward current becomes larger than net outward current. The resulting depolarization becomes self-sustaining and gives rise to the upstroke of the action potential. If net inward current is less than net outward current, no action potential will occur (i.e., all-or-none response). 2. Ionic basis of the nerve action potential (Figure 1.6) a. Resting membrane potential is approximately −70 mV, cell negative. + is the result of the high resting conductance to K , which drives the membrane poten- tial toward the K+ equilibrium potential. + + At rest, the Na channels are closed and Na conductance is low. b. Upstroke of the action potential (1) Inward current depolarizes the membrane potential to threshold. (2) D epolarization causes rapid opening of the activation gates of the Na+ channels, and the Na+ conductance of the membrane promptly increases. he Na+ conductance becomes higher than the K+ conductance, and the mem- (3) T brane potential is driven toward (but does not quite reach) the Na+ equilibrium potential of +65 mV. Thus, the rapid depolarization during the upstroke is caused by an inward Na+ current. (4) T he overshoot is the brief portion at the peak of the action potential when the mem- brane potential is positive. (5) Tetrodotoxin (TTX) and lidocaine block these voltage-sensitive Na+ channels and abolish action potentials. c. R epolarization of the action potential epolarization also closes the inactivation gates of the Na+ channels (but more slowly (1) D than it opens the activation gates). Closure of the inactivation gates results in clo- sure of the Na+ channels, and the Na+ conductance returns toward zero. (2) D epolarization slowly opens K+ channels and increases K+ conductance to even higher levels than at rest. Tetraethylammonium (TEA) blocks these voltage-gated K+ channels. (3) The combined effect of closing the Na+ channels and greater opening of the K+ channels makes the K+ conductance higher than the Na+ conductance, and the membrane potential is repolarized. Thus, repolarization is caused by an outward K+ current. d. Undershoot (hyperpolarizing afterpotential) K+ conductance remains higher than at rest for some time after closure of the The + Na channels. During this period, the membrane potential is driven very close to the K+ equilibrium potential. 0002069201.INDD 10 2/12/2014 10:46:07 AM Chapter 1 Cell Physiology 11 Absolute Relative refractory refractory period period +65 mV Na+ equilibrium potential Action potential Voltage or conductance 0 mV Na+ conductance K+ conductance –70 mV Resting membrane potential –85 mV K+ equilibrium potential 1.0 2.0 Time (msec) Figure 1.6 Nerve action potential and associated changes in Na+ and K+ conductance. 3. Refractory periods (see Figure 1.6) a. Absolute refractory period isthe period during which another action potential cannot be elicited, no matter how large the stimulus. coincides with almost the entire duration of the action potential. + Explanation: Recall that the inactivation gates of the Na channels are closed when the membrane potential is depolarized. They remain closed until repolarization occurs. No action potential can occur until the inactivation gates open. b. Relative refractory period begins at the end of the absolute refractory period and continues until the mem- brane potential returns to the resting level. An action potential can be elicited during this period only if a larger than usual inward current is provided. + Explanation: The K conductance is higher than at rest, and the membrane potential is closer to the K+ equilibrium potential and, therefore, farther from threshold; more inward current is required to bring the membrane to threshold. c. Accommodation occurs when the cell membrane is held at a depolarized level such that the threshold potential is passed without firing an action potential. + occurs because depolarization closes inactivation gates on the Na channels. demonstrated in hyperkalemia, in which skeletal muscle membranes are depol is arized by the high serum K+ concentration. Although the membrane potential is closer to threshold, action potentials do not occur because inactivation gates on Na+ channels are closed by depolarization, causing muscle weakness. 4. P ropagation of action potentials (Figure 1.7) occurs by the spread of local currents to adjacent areas of membrane, which are then depolarized to threshold and generate action potentials. 0002069201.INDD 11 2/12/2014 10:46:09 AM 12 BRS Physiology + + + + – + + + + – – – – + – – – – Figure 1.7 Unmyelinated axon showing spread of depolarization by local current flow. Box shows active zone where action potential had reversed the polarity. Myelin sheath Node of Ranvier Figure 1.8 Myelinated axon. Action potentials can occur at nodes of Ranvier. Conduction velocity is increased by: a. ↑ fiber size. Increasing the diameter of a nerve fiber results in decreased internal resis- tance; thus, conduction velocity down the nerve is faster. b. Myelination. Myelin acts as an insulator around nerve axons and increases conduction velocity. Myelinated nerves exhibit saltatory conduction because action potentials can be generated only at the nodes of Ranvier, where there are gaps in the myelin sheath (Figure 1.8). V. Neuromuscular and Synaptic Transmission A. General characteristics of chemical synapses 1. An action potential in the presynaptic cell causes depolarization of the presynaptic terminal. 2. A s a result of the depolarization, Ca2+ enters the presynaptic terminal, causing release of neurotransmitter into the synaptic cleft. 3. Neurotransmitter diffuses across the synaptic cleft and combines with receptors on the postsynaptic cell membrane, causing a change in its permeability to ions and, conse- quently, a change in its membrane potential. 4. Inhibitory neurotransmitters hyperpolarize the postsynaptic membrane: excitatory neuro transmitters depolarize the postsynaptic membrane. B. Neuromuscular junction (Figure 1.9 and Table 1.2) is the synapse between axons of motoneurons and skeletal muscle. The neurotransmitter released from the presynaptic terminal is ACh, and the postsynaptic membrane contains a nicotinic receptor. 1. Synthesis and storage of ACh in the presynaptic terminal Choline acetyltransferase catalyzes the formation of ACh from acetyl coenzyme A (CoA) and choline in the presynaptic terminal. ACh is stored in synaptic vesicles with ATP and proteoglycan for later release. 2. Depolarization of the presynaptic terminal and Ca2+ uptake Action potentials are conducted down the motoneuron. Depolarization of the presyn- aptic terminal opens Ca2+ channels. 0002069201.INDD 12 2/12/2014 10:46:14 AM Chapter 1 Cell Physiology 13 AChR Action potential in nerve 3 Action potential in muscle ACh 1 5 Na+ K+ Ca2+ 2 4 Motoneuron Muscle Figure 1.9 Neuromuscular junction. ACh = acetylcholine; AChR = acetylcholine receptor. When Ca2+ permeability increases, Ca2+ rushes into the presynaptic terminal down its electrochemical gradient. 3. Ca2+ uptake causes release of ACh into the synaptic cleft Thesynaptic vesicles fuse with the plasma membrane and empty their contents into the cleft by exocytosis. 4. Diffusion of ACh to the postsynaptic membrane (muscle end plate) and binding of ACh to nicotinic receptors + + The nicotinic ACh receptor is also a Na and K ion channel. Binding of ACh to α subunits of the receptor causes a conformational change that opens the central core of the channel and increases its conductance to Na+ and K+. These are examples of ligand-gated channels. 5. End plate potential (EPP) in the postsynaptic membrane Because the channels opened by ACh conduct both Na+ and K+ ions, the postsynaptic membrane potential is depolarized to a value halfway between the Na+ and K+ equilib- rium potentials (approximately 0 mV). The contents of one synaptic vesicle (one quantum) produce a miniature end plate potential (MEPP), the smallest possible EPP. MEPPs summate to produce a full-fledged EPP. The EPP is not an action potential, but simply a depolarization of the specialized muscle end plate. 6. Depolarization of adjacent muscle membrane to threshold Once the end plate region is depolarized, local currents cause depolarization and action potentials in the adjacent muscle tissue. Action potentials in the muscle are followed by contraction. t a b l e 1.2 Agents Affecting Neuromuscular Transmission Effect on Neuromuscular Example Action Transmission Botulinus toxin Blocks release of ACh from Total blockade presynaptic terminals Curare Competes with ACh for receptors Decreases size of EPP; maximal doses on motor end plate produce paralysis of respiratory muscles and death Neostigmine Inhibits acetylcholinesterase Prolongs and enhances action of ACh at muscle end plate Hemicholinium Blocks reuptake of choline into Depletes ACh stores from presynaptic terminal presynaptic terminal ACh = acetylcholine; EPP = end plate potential. 0002069201.INDD 13 2/12/2014 10:46:16 AM 14 BRS Physiology 7. Degradation of Ach The EPP is transient because ACh is degraded to acetyl CoA and choline by acetylcholin- esterase (AChE) on the muscle end plate. One-half of the choline is taken back into the presynaptic ending by Na+-choline cotransport and used to synthesize new ACh. AChE inhibitors (neostigmine) block the degradation of ACh, prolong its action at the muscle end plate, and increase the size of the EPP. Hemicholinium blocks choline reuptake and depletes the presynaptic endings of ACh stores. 8. Disease—myasthenia gravis is caused by the presence of antibodies to the ACh receptor. is characterized by skeletal muscle weakness and fatigability resulting from a reduced number of ACh receptors on the muscle end plate. The size of the EPP is reduced; therefore, it is more difficult to depolarize the muscle membrane to threshold and to produce action potentials. Treatment with AChE inhibitors (e.g., neostigmine) prevents the degradation of ACh and prolongs the action of ACh at the muscle end plate, partially compensating for the reduced number of receptors. C. Synaptic transmission 1. Types of arrangements a. One-to-one synapses (such as those found at the neuromuscular junction) An action potential in the presynaptic element (the motor nerve) produces an action potential in the postsynaptic element (the muscle). b. Many-to-one synapses (such as those found on spinal motoneurons) An action potential in a single presynaptic cell is insufficient to produce an action potential in the postsynaptic cell. Instead, many cells synapse on the p ostsynaptic cell to depolarize it to threshold. The presynaptic input may be excitatory or inhibitory. 2. Input to synapses The postsynaptic cell integrates excitatory and inhibitory inputs. When the sum of the input brings the membrane potential of the postsynaptic cell to threshold, it fires an action potential. a. Excitatory postsynaptic potentials (EPSPs) are inputs that depolarize the postsynaptic cell, bringing it closer to threshold and closer to firing an action potential. + are caused by opening of channels that are permeable to Na and K+, similar to the ACh channels. The membrane potential depolarizes to a value halfway between the equi- librium potentials for Na+ and K+ (approximately 0 mV). Excitatory neurotransmitters include ACh, norepinephrine, epinephrine, dopamine, glutamate, and serotonin. b. Inhibitory postsynaptic potentials (IPSPs) are inputs that hyperpolarize the postsynaptic cell, moving it away from threshold and farther from firing an action potential. are caused by opening Cl− channels. The membrane potential is hyperpolarized toward the Cl− equilibrium potential (−90 mV). Inhibitory neurotransmitters are γ-aminobutyric acid (GABA) and glycine. 3. Summation at synapses a. Spatial summation occurs when two excitatory inputs arrive at a postsynaptic neuron simultaneously. Together, they produce greater depolarization. 0002069201.INDD 14 2/12/2014 10:46:16 AM Chapter 1 Cell Physiology 15 b. Temporal summation occurs when two excitatory inputs arrive at a postsynaptic neu- ron in rapid succession. Because the resulting postsynaptic depolarizations overlap in time, they add in stepwise fashion. c. Facilitation, augmentation, and posttetanic potentiation occur after tetanic stimula- tion of the presynaptic neuron. In each of these, depolarization of the postsynaptic euron is greater than expected because greater than normal amounts of neurotrans- n mitter are released, possibly because of the accumulation of Ca2+ in the presynaptic terminal. Long-term potentiation (memory) involves new protein synthesis. 4. Neurotransmitters a. ACh (see V B) b. Norepinephrine, epinephrine, and dopamine (Figure 1.10) (1) N orepinephrine is the primary transmitter released from postganglionic sympathetic neurons. is synthesized in the nerve terminal and released into the synapse to bind with a or b receptors on the postsynaptic membrane. is removed from the synapse by reuptake or is metabolized in the presynaptic ter- minal by monoamine oxidase (MAO) and catechol-O-methyltransferase (COMT). The metabolites are: (a) 3,4-Dihydroxymandelic acid (DOMA) (b) N ormetanephrine (NMN) (c) 3 -Methoxy-4-hydroxyphenylglycol (MOPEG) (d) 3-Methoxy-4-hydroxymandelic acid or vanillylmandelic acid (VMA) pheochromocytoma, a tumor of the adrenal medulla that secretes catechol- In amines, urinary excretion of VMA is increased. (2) Epinephrine is synthesized from norepinephrine by the action of phenylethanolamine- N-methyltransferase in the adrenal medulla a methyl group is transferred to norepinephrine from S-adenosylmethionine Tyrosine tyrosine hydroxylase L-dopa dopa decarboxylase Dopamine dopamine β-hydroxylase Norepinephrine phenylethanolamine-N-methyltransferase (adrenal medulla) Epinephrine Figure 1.10 Synthetic pathway for dopamine, norepi- nephrine, and epinephrine. 0002069201.INDD 15 2/12/2014 10:46:17 AM 16 BRS Physiology (3) D opamine is prominent in midbrain neurons. is released from the hypothalamus and inhibits prolactin secretion; in this context, it is called prolactin-inhibiting factor (PIF). is metabolized by MAO and COMT. (a) D 1 receptors activate adenylate cyclase via a Gs protein. (b) D 2 receptors inhibit adenylate cyclase via a Gi protein. (c) P arkinson disease involves degeneration of dopaminergic neurons that use the D2 receptors. (d) Schizophrenia involves increased levels of D2 receptors. c. Serotonin is present in high concentrations in the brain stem. is formed from tryptophan. is converted to melatonin in the pineal gland. d. Histamine is formed from histidine. is present in the neurons of the hypothalamus. e. Glutamate is the most prevalent excitatory neurotransmitter in the brain. There are four subtypes of glutamate receptors. Threesubtypes are ionotropic receptors (ligand-gated ion channels) including the NMDA (N-methyl-d-aspartate) receptor. One subtype is a metabotropic receptor, which is coupled to ion channels via a heterotrimeric G protein. f. GABA is an inhibitory neurotransmitter. is synthesized from glutamate by glutamate decarboxylase. has two types of receptors: (1) The GABAA receptor increases Cl− conductance and is the site of action of benzodi- azepines and barbiturates. (2) The GABAB receptor increases K+ conductance. g. Glycine is an inhibitory neurotransmitter found primarily in the spinal cord and brain stem. − increases Cl conductance. h. Nitric oxide (NO) is a short-acting inhibitory neurotransmitter in the gastrointestinal tract, blood ves- sels, and the central nervous system. is synthesized in presynaptic nerve terminals, where NO synthase converts arginine to citrulline and NO. is a permeant gas that diffuses from the presynaptic terminal to its target cell. also functions in signal transduction of guanylyl cyclase in a variety of tissues, including vascular smooth muscle. VI. Skeletal Muscle A. Muscle structure and filaments (Figure 1.11) Each muscle fiber is multinucleate and behaves as a single unit. It contains bundles of myofibrils, surrounded by SR and invaginated by transverse tubules (T tubules). 0002069201.INDD 16 2/12/2014 10:46:18 AM Chapter 1 Cell Physiology 17 A Motoneuron Muscle Sarcomere I band I band Thin filament Thick filament Myofibril Z line M line Z line H band A band B Transverse tubules Sarcolemmal membrane Terminal cisternae Sarcoplasmic reticulum Figure 1.11 Structure of the sarcomere in skeletal muscle. A: Arrangement of thick and thin filaments. B: Transverse tubules and sarcoplasmic reticulum. Each myofibril contains interdigitating thick and thin filaments arranged longitudinally in sarcomeres. Repeating units of sarcomeres account for the unique banding pattern in striated muscle. A sarcomere runs from Z line to Z line. 1. Thick filaments are present in the A band in the center of the sarcomere. contain myosin. a. M yosin has six polypeptide chains, including one pair of heavy chains and two pairs of light chains. b. Each myosin molecule has two “heads” attached to a single “tail.” The myosin heads bind ATP and actin and are involved in cross-bridge formation. 2. Thin filaments are anchored at the Z lines. are present in the I bands. 0002069201.INDD 17 2/12/2014 10:46:37 AM 18 BRS Physiology interdigitate with the thick filaments in a portion of the A band. contain actin, tropomyosin, and troponin. a. Troponin is the regulatory protein that permits cross-bridge formation when it binds Ca2+. b. Troponin is a complex of three globular proteins: Troponin T (“T” for tropomyosin) attaches the troponin complex to tropomyosin. Troponin I (“I” for inhibition) inhibits the interaction of actin and myosin. Troponin C (“C” for Ca ) is the Ca -binding protein that, when bound to Ca , 2+ 2+ 2+ permits the interaction of actin and myosin. 3. T tubules are an extensive tubular network, open to the extracellular space, that carry the depolarization from the sarcolemmal membrane to the cell interior. are located at the junctions of A bands and I bands. contain a voltage-sensitive protein called the dihydropyridine receptor; depolarization causes a conformational change in the dihydropyridine receptor. 4. SR 2+ is the internal tubular structure that is the site of Castorage and release for excitation– contraction coupling. has terminal cisternae that make intimate contact with the T tubules in a triad arrangement. 2+ 2+ membrane contains Ca -ATPase (Ca pump), which transports Ca from intracellular 2+ 2+ fluid into the SR interior, keeping intracellular [Ca ] low. contains Ca bound loosely to calsequestrin. 2+ contains a Ca release channel called the ryanodine receptor. 2+ B. Steps in excitation–contraction coupling in skeletal muscle (Figures 1.12 and 1.13) 1. Action potentials in the muscle cell membrane initiate depolarization of the T tubules. 2. Depolarization of the T tubules causes a conformational change in its dihydropyridine receptor, which opens Ca2+ release channels (ryanodine receptors) in the nearby SR, caus- ing release of Ca2+ from the SR into the intracellular fluid. 3. I ntracellular [Ca2+] increases. 4. C a2+ binds to troponin C on the thin filaments, causing a conformational change in troponin that moves tropomyosin out of the way. The cross-bridge cycle begins (see Figure 1.12): a. A t first, no ATP is bound to myosin (A) and myosin is tightly attached to actin. In rapidly contracting muscle, this stage is brief. In the absence of ATP, this state is permanent (i.e., rigor). b. ATP then binds to myosin (B) producing a conformational change in myosin that causes myosin to be released from actin. c. Myosin is displaced toward the plus end of actin. There is hydrolysis of ATP to ADP and inorganic phosphate (Pi). ADP remains attached to myosin (C) d. Myosin attaches to a new site on actin, which constitutes the power (force-generating) stroke (D) ADP is then released, returning myosin to its rigor state. e. The cycle repeats as long as Ca2+ is bound to troponin C. Each cross-bridge cycle “walks” myosin further along the actin filament. 5. Relaxation occurs when Ca2+ is reaccumulated by the SR Ca2+-ATPase (SERCA). Intracellular Ca2+ concentration decreases, Ca2+ is released from troponin C, and tropomyosin again blocks the myosin-binding site on actin. As long as intracellular Ca2+ concentration is low, cross-bridge cycling cannot occur. 6. Mechanism of tetanus. A single action potential causes the release of a standard amount of Ca2+ from the SR and produces a single twitch. However, if the muscle is stimulated repeat- edly, more Ca2+ is released from the SR and there is a cumulative increase in intracellular [Ca2+], extending the time for cross-bridge cycling. The muscle does not relax (tetanus). 0002069201.INDD 18 2/12/2014 10:46:44 AM Chapter 1 Cell Physiology 19 Actin filament – + Myosin head Myosin filament A – + – + ADP ATP D B – + ADP Pi C Figure 1.12 Cross-bridge cycle. Myosin “walks” toward the plus end of actin to produce shortening and force generation. ADP = adenosine diphosphate; ATP = adenosine triphosphate; Pi = inorganic phosphate. C. Length–tension and force–velocity relationships in muscle Isometric contractions are measured when length is held constant. Muscle length (preload) is fixed, the muscle is stimulated to contract, and the developed tension is measured. There is no shortening. Isotonic contractions are measured when load is held constant. The load against which the muscle contracts (afterload) is fixed, the muscle is stimulated to contract, and shortening is measured. 1. Length–tension relationship (Figure 1.14) measures tension developed during isometric contractions when the muscle is set to fixed lengths (preload). Action potential