Molecular Biology Lecture Notes PDF
Document Details
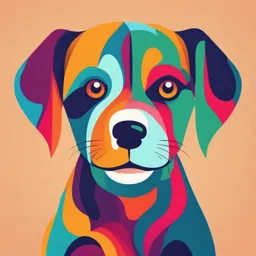
Uploaded by .keeks.
Marian University
Tags
Summary
Lecture notes on molecular biology, specifically focusing on genetic material movement, transposons, and their relation to immune responses and gene therapy. The lecture also touches on fundamental concepts of DNA and RNA.
Full Transcript
Hey, it is one o'clock and time to get started with this fun material. This is the last section of new material. Whatever we don't get through, I will do like a mini recording of, it should only be at most a couple of slides, but hopefully we get through it just fine. This means that everything asso...
Hey, it is one o'clock and time to get started with this fun material. This is the last section of new material. Whatever we don't get through, I will do like a mini recording of, it should only be at most a couple of slides, but hopefully we get through it just fine. This means that everything associated with exam three you have access to. So no more new content, just practice. Make sense? So our flipped classroom will focus on the practice for the gene code prerecorded material. All right, so this slide was one that came up while I was kind of in the zone on transcriptional regulation and I completely bypassed it. Not my finest moment. So I want to start here today and get us through what my expectations for VDJ recombination are and where we're sort of focused. And one of the things that I want to talk about, got a place to sit today. One of the things that I want to talk about is the idea of change in your lifetime. Change in your lifetime is possible. Yes, we'd like to believe that we are capable of learning from our mistakes, of course, right? Your DNA is capable of change in your lifetime and that's essential to many critical processes. What if you could only make five antibodies? Five versions of five specific antibodies, that's it. One, two, three, four, five. What would that mean in terms of immunological function? Wouldn't be very good, right? You would not be capable of the adaptation necessary to respond to incoming antigens. You would not be capable of eliciting immune responses in an adaptive manner. So it turns out that you do in fact have sequences of genetic material that are capable of movement. And we refer to these generically as transposons. This is material that can move or transpose from one place to another. This is actually really great because these transposable elements, these portions of the material that enable this movement helps us to increase the genetic variation of key genes. You know that you have a constant region and a variable region for antibodies, correct? And those regions are actually determined by the code. So instead of processing at the RNA level like we do for many things, we can actually process at the DNA level to make those determinations. And this gives us two levels of potential for change. It gives us the ability to make far more products than we would if we did not have this system. So for this, we are going to regulate this conceptually in the same way that we regulate other genes. What is the predominant mode of regulation for eukaryotic genes? Transcriptional regulation. We are going to control whether we transcribe the material necessary for transposition. Am I actually going to make the things that will enable this material to move or not? If I make it, the material can move. If I don't, it cannot. And so we actually use these concepts in treatment. We exploit this ability for DNA to move around as part of our gene therapy to improve phenotypes and physiological outcomes. So we are going to focus on the nature of transposition. And essentially, in order for material to move, it's gotta be pulled out, find a new place, and enter. What does pulling out material mean you have to do? You gotta cleave that backbone, right? You gotta cleave out the sugar so that you can take this material and move it to a new location. What does that sound like from a perspective of error? You're breaking things. You're creating breaks. You are taking that backbone, and you are taking away the stability of it. So if you do that without properly regulating where you're doing those cuts, what could you do? You could literally break the middle of a gene. You could break a promoter. These are those structural rearrangement connections, right? We talked about chromosomal-level structural rearrangements as being part of normal recombination, but these are some of the places where repair and the ability of DNA to change itself comes into play for those as well. We can actually cause deletions. We can cause positional effect or insertional mutagenesis when we put the material back in, but ultimately we are changing the gene code which has the potential to change what we make or if we can even make it. Does it make sense? There's two classes of these. What I'm really talking about right now is really more DNA to DNA, the class two, but you can have RNA material that is designed to be reverse transcribed into DNA material to enter the genome. Viruses very similarly use a process to this to get their stuff into our genome when they are capable of this sort of insertion. We are gonna focus on the idea of recognition, induction of breaks, and then you've gotta ligate that. What happens if you don't ligate it? Your break is there. Do we have mechanisms to repair breaks, to maybe catch it later? Yes, but what if you don't? That could stall transcription, it could stall translation, but then the most important part which gives us that tie-in that I talked to you about with HPV, insertional mutagenesis. If I insert this material in the middle of a gene, in the middle of a promoter, in the wrong place, I disrupt what was originally there. And when a virus like HPV does this, we actually lose regulation. And some of the genes specifically affected by certain HPVs are linked to cancer potential. They're literally oncogenic in nature or you lose a tumor suppressor depending. So insertional mutagenesis has direct consequences for cellular behavior. So this is the callback to what you've done before where you talk about the idea of variable and constant segments. And I know you had the rearrangement in the organization and you did all this before. I am not asking you to remember the specifics of this event. What I want to talk about is the idea of how we can take that specific example and apply it more broadly. Rag-mediated transposition. What does that sound like? It sounds like a molecule called rag is responsible for transposition, right? Rag is recombination activating genes. They actually are responsible for the concept of transposition and it's going to be what enables you to cut and insert material. So our rag one is an endonuclease. What do nucleases do? Cut sugar backbones, right? So we are going to cleave and we're going to function with rag two to actually make sure that we can splice out material. So this connects to our VDJ because it's going to be molecules associated with that action as well. We use double-stranded break repair to fix what we do. So I'm going to cut material out, fix the section I just cut from and I'm going to take that material and move it to a new place. If I don't want it in a new place, I'm going to target it for degradation. I'm going to chop it up with more exonuclease activity and more nucleases or I'm going to have signal sequences that now allow me to put it someplace new. And so if we think about this from that perspective of VDJ recombination, I am literally enabling things to come together using processes like non-homologous end joining to create the new piece. And so our big tie into this is all of our repair processes, when they go wrong, have the potential to cause damage. I feel something wrong. I stick two ends together. That's bad. Especially if it's two sister chromatids at their telomeres where I go from having two nice sticks to a weird fusion. Non-homologous end joining can do that. And essentially all the things that we talk about in terms of mutation can be developed from aberrant natural processes. Transposition is supposed to happen. There are specific situations in which we want it to happen. But if we mutate RAG1 or RAG2, we can impair this and cause this to happen at inappropriate times, inappropriate locations. And if we then fuse those, we can get deletions. We can get other losses or even gains. We can create fusion proteins from fusion genes because we stuck two things together that were not supposed to go together. And that dysregulates both of those proteins as a result. And there are direct ties to these actions in cancer. It's important to note that specifically RAG1 and RAG2 deficiencies are associated with errors in immune system maturation. Specifically T and B cell development. So does that sort of put transposition in the context of what we want and sort of help us do that? Here was the specific example you used, but broadly because RAG1 and RAG2 are carrying out this activity of removal. And then we're using our repair mechanisms to fix that removal. We have a direct line toward potential damage and increasing levels of damage at that. Make sense? Is that a little bit easier than what you thought I was gonna do? Because we were very, very vocal in our displeasure at VDJ recombination. So are we good now? What my expectation for this is? What happens if we pull out a transposon and stick it in the wrong place? What is that called? Insertional mutagenesis and what's the consequence? Long answer? Depends, right? What if it's in the middle of a promoter? I'm gonna affect transcription. What if it's in the middle of a coding region? Is that another? Depends. It's in the middle of an intron, doesn't affect splicing. Not a lot. Middle of the coding region, like an insertion of an odd number of bases outside the set of three. Frame shift is described there, right? Make sense? Okay, so let's talk about my favorite part of this because I think it brings a lot of things full circle and the one thing that makes us special, RNA processing. We're gonna talk about all the different parts of RNA, it's not the one thing that makes us special, but we've been talking a lot about prokaryotes and this is definitely eukaryotes, right? We process, process, process. Why do we do that? What's the reason? We're not on this yet, I'm asking a general question. Why do we process our material? What's different about our genes from prokaryotes? They're split. There is material in between the coding regions of our genes. Prokaryotes have colinear, I know it's coming up on a slide but I wanna make sure we're starting this at the beginning. Prokaryotes have colinear material, we have split genes. If your material is split, you must put it back together before you can use it. Why wouldn't we want to do that always at the DNA level? Why do that first part and this part go together so well? Why wouldn't we just use transposition to rearrange every product? Because transposition is not really reversible and once it's done, that's that. So is it okay to do that to a colony of B cells? Yeah, a colony of B cells because that's gonna give you different antibodies produced from that clonal growth, right? Do we wanna do that to all the B cells that exist? Why not? Because that variable region that we were so dependent on just became what? Constant, you just made them all the same. So transposition doesn't make sense as a permanent means of giving you variation from a coding portion. RNA processing does. Splice and alternative splicing makes sense for giving us multiple forms from a single coding region. In addition to that, once we've made RNA, we can do more to it. We talked about base deamination as a form of damage. It exists as a form of damage because we can use it to edit RNA. RNA editing at the sequence level gives us variation. By changing the literal bases of the mRNA, we can get a different product. And so we have a very direct example of using enzymes that cause the amination, that remove those amino groups to create different forms of apoprotein A100. I'm sorry, B100. I always forget that. Sorry about that. When we have the full form, we have different activity than when we have the truncated form. Rather than trying to control that at the level of transcription or trying to edit the DNA, which is an irreversible process, I can make a simple change to a base of the mRNA and get these two different products as needed. So now all I have to do is control the amination. Seems a lot easier, doesn't it? So I can control that in time and space. This is an important concept because it also sort of highlights what happens when transcription doesn't do its job as well as it should, when the RNA polymerase isn't as accurate as it should be. Because it kind of gives us a framework for maybe we can fix some RNA molecules. Even if we're not doing the same editing that we think of classically with repair, there are some things that we can do to an mRNA molecule that edits it in a way that helps to maintain or restore it or create new products. And this all gives us increasing variation without changing the DNA. It enables us to not be limited, to not have every cell type behave the same way. Processing is dependent on physiological need. What products I make is going to be dependent on what I need in that cell type at that time. Whether I undergo alternate splicing or not, it's going to be related to what does the cell need to be able to do. Because what is the point of mRNA? To encode for protein. We control at what level predominantly, GNEC, at the level of transcription. Regulation is predominantly at the level of transcription. So processing helps to ensure that we're getting the right product to make protein from. We do see some level of processing all the way down to prokaryotes. So even though they don't explicitly have introns in the same sense that we have, you can actually see them have some sequences that they remove and mess with a little. It's not going to be like us where we can't even make the full thing without removing these large segments in the nucleus, but they will do some processing. They will have some cleavage events. And so we refer to that material as intron-like, because it's really about increasing functionality as opposed to necessary removal to make sure the molecule can be created. Does this make sense? It's kind of a subtle distinction, but the idea here is that sometimes things don't come out exactly right, even though they're collinear, they're sometimes material. We don't need in the end, and so we're going to process and remove that all the way down to prokaryotes. Eukaryotes have those split genes. So every single mRNA molecule is processed. Every single one. Doesn't leave a nucleus without that processing. This is not new information, right? Exons exit the nucleus, introns stay in. This is generally referred to as splicing. The idea of removing material and fusing together your oculoploid. This is transposition, but now we're talking about an mRNA module. There is no such thing as junk DNA. So then what is an intron? An intron is material that helps us identify where splicing should take place. Just because you're getting rid of it does not mean that it doesn't have sequence importance. You're getting rid of it because the product you need to make is the fusion of one and two instead of one and three or one and four. Alternate splicing, which is the last two slides of this, so it'll come full circle, means that the intron is going to be playing a role in deciding what goes. In addition to removing introns, we have other processing. We have capping and polyadenylation of the tail. Capping is critical to overall function and stability of an mRNA molecule, as is the poly-A tail. Because the five prime cap can vary widely, we do get different levels of half-life, meaning how stable a molecule is. Methyltransferase activity is what does a cap. A cap is adding a methyl guanine, so we're gonna have a transfer of a methyl to one location to another. We have variable structure for this across all of our organisms, but we do have a set structure for mammals. Our main form is this seven methyl guanosine, which we link to whatever the first transcribed nucleotide using a triphosphate bridge. It's a lot about chemistry there, but ultimately what we're talking about is we're gonna move this material right here onto the five prime and right near the very first letter, very first nucleotide. Doesn't matter what that first nucleotide is, the cap is always going to the same place, and it is a seven methyl guanosine. The methyltransferase that typically does this for us is the capping, C-A-P-A-M. And so you can see that we have the methylated position, a triphosphate bridge, and then our sequence. Different caps, so we still always have that, funny, but like different capping material, different first base, so that total cap of that first nucleotide, the triphosphate bridge, and the seven methyl guanosine has different stability. What do we mean by stability? How did we apply it for DNA? Half-life melting temperature concept, right? Higher the temperature necessary to unzip that material. For our RNA, the half-life concept is how long the molecule stays stable for translation. Stronger caps equal longer half-life. Weaker caps equal shorter half-life. Specifically, because we are protecting from nuclease activity, a better cap, harder to cut. Worse cap, easier to cut up. Once we cut up an mRNA molecule, it's done. Can I make a protein from that? No, because we need that cap for the small subunit of the ribosome to associate. Without the cap, translation cannot occur for us. It's important to note that not just mRNA gets processed. What did Paul II do if I added an anti-termination factor to it? It kept going and it made what? SNRNAs. SNRNAs and MRNAs have very similar elements that regulate them. We have what are referred to as upstream and downstream elements that help to control their processing. Both SNRNA and mRNA will be processed. SNRNAs don't just come out of the box ready to go. They've got to be cleared. They've got to be activated. They're our genes. What are our genes? Are they co-linear or they're split? So it doesn't matter what gene we're talking about. Some processing is going to have to happen. So some of the differences are what those specific elements are. So we call DSEs or distal sequence elements. Ds in an SNRNA are like an enhancer. What's the job of an enhancer? Enhance transcription by helping bind factors that increase RNA polymerase affiliation with the promoter, affinity for the promoter, helping to land RNA polymerase. So these DSEs are helping to ensure that RNA pol II is coming in and doing its job. The proximal sequence element is like a promoter. So we're trying to make sure, the DSE is trying to make sure the polymerase lands at the right place, the PSE. Since this is often a continuation of the activity of pol II, these elements are really playing more of a role when we're not continuing, but what? Landing for the first time without the mRNA, right? That still has to happen. Sometimes, SRNA is made even when you haven't made the mRNA that comes right before it, right? Can we imagine a scenario where that might be the case? When we don't need the mRNA, but we do need the SRNA, the SNRNA? Why would we have a situation where we can continue past the mRNA? Sorry, review, because I lost you for a second. We have mRNA. We've just made it. Why are we keeping the polymerase going? Because we want that SNRNA, and here's the connection, what we're talking about today. That SNRNA might be playing a role in processing that mRNA. Does that make sense? So just because there are times when we are linked doesn't mean we are always linked. And so these distal sequence elements, the enhancer-like and promoter-like sequences allow us to also transcribe that gene when we're not transcribing the material that came right before it. So I just want to remind us that we can turn these genes on without having transcribed an mRNA right before it. RNA PUL2 can do these even if they haven't made an mRNA right before it. It's still a gene. It can still be turned on and regulated. That's the point. They have similar sequences, but they're not exactly the same. SNRNA are very special. Make sense? What are SNRNAs? Small, nuclear, what do they do? I know we haven't quite gotten to those slides, but what category of RNA do they fall into? Structural. Why are they structural? Because they contribute to generating or ensuring critical structures or processes. They are part of the spliceosome. And so the comparison I'm trying to draw here is to show you that mRNAs and SNRNAs are incredibly similar. And they are companion concepts because I cannot process an mRNA molecule without SNRNA. But there are times when I'm going to want the SNRNA regardless of the mRNA. So just remembering, yes, transcription can create these as an extension of mRNA, but it can also just make them. They're still genes. Genes can be transcribed independently. And these elements are how? SNRNA are a critical component of the spliceosome. Specifically, they associate with proteins to generate SNRNPs. How do I determine what sequence of bases exists? I am looking at a sequence of bases. I am, oh, I don't know, telomerase. How do I determine what I'm going to do? What does telomerase do? It elongates the ends of chromosomes. It elongates telomeres. And it has two components. What are they? A template and an enzyme. Why do I have the template so I know what to make? How do I know where to cut? How do I know where to process something? How do I identify that's the right location? I have to be able to read the sequence. I have to be able to say, this is the right spot. And some of the ways in which we read the sequence is to have complementary bases to that sequence. The spliceosome is made up of SNRNAs because the SNRNAs locate the splice sites. They say, this is the right sequence to cut. This is the processing that I wanted to highlight. We are a gene. We are a gene. We are a eukaryotic concept. So there's going to be processing. But what's unique about SNRNA processing is that a mixture of outside the nucleus and inside the nucleus processes. So we actually export and then reimport. Why does this make sense? Why would it make sense to kick something out and then bring it back in? To regulate it. To make sure that you are only bringing in the pieces, the SNRNAs that match the splicing you want to do. So having a process that crosses that lovely compartment gives us the ability to compartmentalize regulation. So now we have splicing. We've set all the pieces in motion. We've got all the players. We've got our mRNA with all its introns. We've got our SNRNA to be able to identify sequences. Now we're going to splice. We're going to cut things up and shove them together the way we want. SNRNPs are a mixture of SNRNA so pieces of RNA that give us the nucleotide sequences and proteins. The SNRNP is a functional unit for splicing. It is going to determine where we splice. Different SNRNAs give us different splice sites. Are all introns created equal? Do they have all the identical same sequence? No. So we need more than one SNRNA to be able to do this. So if we're looking for a distinction between SNRNA and SNRNP because so many close letters are so much fun, SNRNA, sequence recognition, but needs the protein for any sort of catalytic or action. So activity requires the full SNRNP, but the literal sequence is the SNRNA. SNRNP, functional unit SNRNA sequence. We have steps that are recognition, processing, actual catalytic activity, and then sealing the backbone. It's important to note that the pieces we remove, we don't want hanging around. They have to be processed and eliminated. So they also, the introns that we remove, they also form critical structures. U1, U2, and U5 are working together as part of recognition. We're going to talk about this in a little bit more detail in a second. But let's start with that intron. I've got a piece of DNA I don't want anymore. Sorry, this is RNA. I've got a piece of, well, yeah, no, DNA works, too. I've got a piece of anything I don't want anymore that is a nucleotide-driven sequence. Sorry, this is where my brain was going. How do I get rid of nucleotides? I need nucleases. I've got to cut them up, right? How do I prevent myself from just cutting up everything? So I have the mechanism in place to cut up bits of DNA or cut up bits of RNA. How do I control where and when I do that? Well, I can control whether or not I provide those nucleases, right? I can control at the level of nuclease. But what if they're constitutively expressed and they're just always there? How do I control so I don't destroy every mRNA molecule all the time? Well, I've got capping and stabilization. But the other side of that is I can label things for destruction. I can have a system where I label to say that should be destroyed rather than just protecting from destruction, right? I can have both negative and positive indicators. The lariat structure that we form after we make an initial splice is like a flag that says, come to me up. I'm right here. That structure is critical. So we have our first recognition. Our SNRNA is going to align with this splice site. And it's going to say, this is where I cut. And so we're going to have a more complex process we're going to get to in a second that's going to label that cut. But the initial cut, that initial nucleus activity, is going to enable bending back of that material onto itself from a splice site to a branch site to create this right here. And this right here is called the lariat. And this little circle helps to say, come destroy this. That opening I've created when I cut first this end and then the three prime end, when I do that cut, I then have to pull those pieces together. That's going to be a fusion event. So I'm going to fuse the end of this exon with the beginning of this one. But this removed structure is now wearing a giant sign, a giant flag that says, come destroy me. And it's going to be very quickly chopped up. Why is it beneficial? Why is cutting something up into its base nucleotides very quickly a highly beneficial concept? Doesn't it restore our pool of nucleotides? Meaning we don't have to re-synthesize all those nucleotides. We just now have those readily available in the nucleus. Choosing which splice sites gives me the ability to have multiple products. So here it is, assembly and activity. Prokaryotes don't have what? Membrane bound organelles. So they don't have compartments. We have compartments. But whereas our prokaryotes had transcription and translation coupled, we have transcription and processing coupled. And so a lot of this processing can actually begin as transcription is taking place. So as soon as we have that 5' end coming out, we can start capping it and we can start processing it. At the site of transcription is where assembly is going to often occur. And I say often because there is evidence of times when it doesn't, but often we are coupling these processes. We are going to bring in the recognition molecules. We're going to recognize the first location. And this is a process that more recent updates have identified processing of the process occurs. So to simplify this as much as is possible, we are essentially going to create identification, like we're going to see it, create the molecule that's going to do the cutting, determine where those cuts are going to take place, activate that complex, cut the material, fuse the mRNA, and be done. Does that make sense? As simplified as I can. Identify the spot, cut the material out, make sure that the material we cut out gets destroyed, and make sure the pieces we left together get stuck together. We need to make sure that we are choosing the desired exons. If we're going to be picking one and three, we need to make sure those are the pieces. And much like all of our processes, we want to use a system of recycling so that we're more efficient. So when we release these SNR and P's, they can go on to do the next intron, or they can be part of the next process where they are needed. Let's do this from the more complicated piece now. We are going to have you one and you two finding our spot. This is complex A. Complex A is referred to as the pre- splice zone. It's like planting a flag of here. Here we go. This is where we're starting. We are then going to bring in U4 slash 6, because they work really, they're a companion at this point, and U5. This transitions us from complex A to complex B. And as we're going to see in a second, complex B gets processed. But we now have complex B. We have helicase activity here. Where helicase is needed before? DNA replication. So what is a helicase doing here? This is single-stranded RNA. It's helping to ensure that we are open and in a confirmation for cleavage. We are making sure that this material isn't folding. Complinary base pairing in RNA creates folds. It creates bending. Helicase activity is important to ensuring we keep the confirmation necessary to cleave. We want to be able to make that lariat, but we need the other part to be accessible. So once we have complex B, we process it. And in this case, we're releasing four and one. So one and two recognized. Now we have four, five, and six coming into play. And we're releasing one and four. Now we are complex C. And we are catalyzing. So we have catalysts. Complex C is now fully formed. And we're going to rearrange complex C, because why wouldn't we? And now we have the post-spliceosomal complex. The rearrangement helps to move the material into the proper orientation so that exons can be fused and introns get out, because it's two cuts. The catalytic subunit is the only subunit that can actually begin cutting. So we form complex B, and we become catalytically active or capable of catalysis. And after we make that first cut, we transition. And now complex C can help cut the other end. We are going to remove two, five, and six to be able to reuse them. How do we actually pull out that spliced material? Is helicase PRP 2, 2, or 22? Make sense? So now we have a lariat we can get rid of, an intron that's gone, and we have a complex. Well, of course, science would make things harder. Processing of complex B is a new thing. What we essentially have is our original thought process was we're identifying the intron, and it's really the intron that matters. And it turns out that some of the recognition matters in terms of the exon we want. But that makes sense in the world of alternate splicing. So we will actually form the spliceosomal complexes potentially in an exon-defined way. So basically, everything I just told you of identify the first intron cut, we are updating to understand that maybe it's also an exon-driven process. And so the evidence of this is that we will have complex B forming initially around an exon and moving to the intron. So what do I want you to know about this? That we do have those steps. We start out at complex A, we become complex B, we have catalysis, we have complex C, and we have to release components in SNR and P's along the way, right? That step-wise process isn't changing. So I'm not as concerned if you identify where it lands, but that we do understand that complex B will be processed. It will have pre and post behaviors because we have identified that there is regulation of it and there is molecular changes to it. There are molecular changes to it. So I realize this is a lot of words to say that we actually have multiple states for complex B and for splicing. And so this is hopefully hitting home the message of we are still learning this stuff. What we do know is that recognition is a carefully regulated process that involves multiple steps and without the corresponding SNR and As generating the corresponding SNR and P's, we don't get splicing. And we certainly don't get site-specific splicing. Does that make sense? I know that was a lot. Okay, so if we are looking for a summary, this slide gives me all the details in bold that are really the goals that I want you to pull away from there. And this slide just sort of tells us that even this year, we are updating that information. Does that make sense? So put a big old X through this. I just want you to understand that complex B is processed. Okay? And this is something I've been talking about this whole time, alternate splicing. And so if we take those recognition roles and we tie it to an exon-defined process, we can now see why we would choose exons 1 and 5 instead of exons 1, 2, 3, 4, 5. This gives us even more variation from our genes because we can have one mRNA that we can process multiple different ways. Alternate splicing, both at the DNA and RNA level, is incredibly important to making us the complex organisms that we are. We can have the same genes, but handle them differently as a result of variation. So processing level regulation. What's our main means of regulation? Transcription. Here is another level, the transcript. And then the final level is translation. So transcription, then how we process it, then how we translate it. Make sense? How we actually regulate alternative splicing while tied to spliceosome assembly can also be controlled by what are called regulatory elements or splicing regulatory elements that include both cis-acting and trans-acting elements. So our cis-acting regulatory elements helps you choose a splice site. The trans-acting factors are what are playing a contributing role to yes and no answers. So localization and then, yeah, maybe. Or, no, not really. So if I'm looking for what I want you to take away from this slide, because I'm trying to do that free as much as possible, is we're going to see these molecules of splicing recognition being regulated by other sequences. So just because I hit that SNRNP on there, there's going to be factors within the genome itself, within the mRNA that are encoded in the genome, that are going to control yes and no to this process. So splicing is incredibly complicated, and we're still learning about it. So why do we care about splicing? You're going to be doctors, right? You're not going to have to go in and splice mRNA, right? We care because errors in these processes correlate with human disease. If I cannot properly splice material, that's catastrophic. If I am constantly unable to splice or create a specific splice variation, I'm going to lose function of that product. And it turns out there are a lot of splice variation pathogenic variants where I either create a new variant or I cannot splice in that way, and it causes or directly correlates with the development of a specific disorder. Does that make sense? So now that you know all the processes of molecular genetics, we can spend all of next block only talking about human disease. So these will pop up at the start of our quick review. I've got a couple of sets of questions because we are almost out of time, and I am determined to stop on time today. So do we have any questions about what we did today? Okay, so we do not have class on Friday because of campus mass from 1 o'clock to 2 o'clock. We will have class on Monday, which is our flipped classroom session, to practice all these things prior to the review on next Wednesday. Questions, concerns? Okay, if you have any questions, I am available over email. Have a great day.