Cellular Respiration: Overview of Energy for Life (PDF)
Document Details
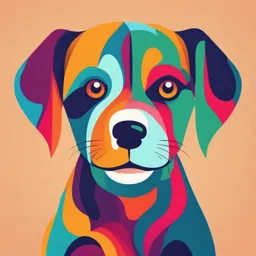
Uploaded by MatureAphorism9471
Tags
Summary
This document explores cellular respiration and photosynthesis. It details the processes in organisms, how energy is generated and used in cells through glycolysis, the citric acid cycle, and oxidative phosphorylation. The role of ATP and the significance of these processes in life are also covered.
Full Transcript
Life requires energy. Figure 6.1 illustrates how photosynthesis and cellular respiration together provide energy for living organisms. In almost all ecosystems, that energy ultimately comes from the sun. In photosynthesis, the energy of sunlight is used to rearrange the atoms of carbon dioxide (CO2)...
Life requires energy. Figure 6.1 illustrates how photosynthesis and cellular respiration together provide energy for living organisms. In almost all ecosystems, that energy ultimately comes from the sun. In photosynthesis, the energy of sunlight is used to rearrange the atoms of carbon dioxide (CO2) and water (H2O), producing organic molecules and releasing oxygen (O2). In cellular respiration, O2 is consumed as organic molecules are broken down to CO2 and H2O, and the cell captures the energy released as ATP. Photosynthesis takes place in some prokaryotes and the chloroplasts of plants and algae. Cellular respiration takes place in many prokaryotes and the mitochondria of almost all eukaryotes—in the cells of plants, animals, fungi, and protists. In all energy conversions, some energy is lost as heat. Life on Earth is solar-powered, and energy makes a one-way trip through an ecosystem. Matter, however, is recycled. The CO2 and H2O released by cellular respiration are converted through photosynthesis to sugar and O2, which are then used in respiration. These processes are fundamental illustrations of the theme of ENERGY AND MATTER. Respiration—often used as a synonym for “breathing”—refers to an exchange of gases: An organism obtains O2 from its environment and releases CO2 as a waste product. Breathing and cellular respiration are closely related. As the runner in Figure 6.2 breathes, her lungs take up O2 and pass it to her blood. Her bloodstream carries the O2 to her muscle cells, where it is used in cellular respiration to produce ATP and power her muscle cells. During the chemical conversions of cellular respiration, the inhaled oxygen atoms become part of the water in her cells (see the equation for cellular respiration at the bottom of the figure; oxygen is a reactant and water is a product). Notice that CO2 is a waste product in the equation summarizing cellular respiration; the atoms in CO2 originate in glucose in this reaction. The bloodstream carries CO2 from her cells to her lungs, where it will be exhaled into the environment. Indeed, breathing and cellular respiration are tightly linked. Breathing and eating provide the reactants needed for cellular respiration—the process that generates ATP for cellular work. The chemical equation in Figure 6.3 summarizes cellular respiration. The simple sugar glucose is the fuel that cells use most often, although other organic molecules such as fats, proteins, and complex carbohydrates can also be “burned” in cellular respiration. The equation tells us that the atoms of the reactant molecules C6H12O6 and O2 are rearranged to form the products CO2 and H2O. In this exergonic (energy-releasing) process, the chemical energy of the bonds in glucose is released, and some are stored (or “banked”) in ATP while the rest is released as heat. Cellular respiration captures about 34% of the available energy originally stored in glucose. This may seem inefficient, but, as a comparison, the average automobile engine converts only about 25% of the energy in gasoline into mechanical energy. In addition, as you learned in the chapter introduction, the heat released in cellular respiration helps you maintain your constant body temperature of 37°C, or 98.6°F. The ability to maintain a constant body temperature is critical to the survival of many animals. How great are the energy needs of a cell? If ATP could not be regenerated through cellular respiration, you would use up nearly your body weight in ATP each day. To demonstrate how much energy your body needs daily, let’s next consider the energy requirements for various human activities. Your body requires a continuous supply of energy just to stay alive—to keep your heart pumping and to keep you breathing. Your brain especially requires a huge amount of energy; its cells burn about 120 grams (g)—a quarter of a pound!—of glucose a day, which accounts for about 20% of total energy consumption. Maintaining brain cells and other life-sustaining activities uses as much as 75% of the energy a person takes in as food during a typical day. Above and beyond the energy you need for body maintenance, cellular respiration provides energy for voluntary activities. The energy units are kilocalories (kcal), a measure of the quantity of heat required to raise the temperature of 1 kilogram (kg) of water by 1°C. (The “Calories” listed on food packages are kilocalories, usually signified by a capital C.) The values shown do not include the energy the body needs for its basic life-sustaining activities, which may range from 1,300 to 1,800 kcal a day. This energy requirement is known as your basal metabolic rate (BMR). The U.S. National Academy of Sciences estimates that the average adult needs about 2,200 kcal of energy per day, although the number varies based on age, sex, and activity level. A balance of energy intake and expenditure is required to maintain a healthy weight. Now we begin the study of how cells liberate the energy stored in fuel molecules (carbohydrates, proteins, and fats) to produce the ATP used to power the work of your cells and thus the activities of your body. During cellular respiration, electrons are transferred from glucose or other organic fuels to oxygen, releasing energy. Oxygen attracts electrons very strongly, and an electron loses potential energy when it moves to oxygen. If you burn a cube of sugar, this electron “fall” happens very rapidly, releasing energy in the form of heat and light. Cellular respiration is more controlled—more like stepping down an energy staircase, with energy released in small amounts that can be stored in the chemical bonds of ATP. The transfer of electrons from one molecule to another is an oxidation-reduction reaction or redox reaction for short. In a redox reaction, the loss of electrons from one substance is called oxidation, and the addition of electrons to another substance is called reduction. A molecule is said to become oxidized when it loses one or more electrons and reduced when it gains one or more electrons. (It might be helpful to remember OIL RIG: Oxidation Is Loss, Reduction Is Gain.) Because an electron transfer requires both a donor and an acceptor, oxidation and reduction always go together. In the cellular respiration equation, you cannot see any electron transfers, but you do see changes in the location of hydrogen atoms. These hydrogen movements represent electron transfers because each hydrogen atom consists of an electron () and a proton (hydrogen ion, or ). Glucose (C6H12O6) loses hydrogen atoms as it is oxidized to CO2; simultaneously, O2 gains hydrogen atoms as it is reduced to H2O. The movement of electrons from glucose to oxygen is an exergonic (energy-releasing) process. The energy released is harnessed by the cell to make ATP. An important player in the process of oxidizing glucose is a coenzyme called NAD+, which accepts electrons and becomes reduced to NADH. NAD+, which stands for nicotinamide adenine dinucleotide, is an organic molecule that cells use to shuttle electrons in redox reactions. An enzyme called dehydrogenase strips two hydrogen atoms from the organic fuel molecule and transfers two electrons and one proton to its coenzyme NAD+, reducing it to NADH. The other proton is released into the surrounding solution. (NADH is represented throughout this chapter as a light brown box carrying two blue electrons.) Cellular respiration consists of a sequence of many chemical reactions that we can divide into three main stages. In prokaryotic cells that use aerobic respiration, these steps occur in the cytosol, and the electron transport chain is built into the plasma membrane. Stage 1: Glycolysis (shown with a teal background throughout this chapter) occurs in the cytosol of the cell. Glycolysis begins cellular respiration by breaking glucose into two molecules of a three-carbon compound called pyruvate. Stage 2: Pyruvate oxidation and the citric acid cycle (shown in shades of orange) take place within the mitochondria. Together, pyruvate oxidation and the reactions of the citric acid cycle complete the breakdown of glucose to carbon dioxide. Thus, the CO2 you exhale is generated in the mitochondria of your cells during this second stage of respiration. As suggested by the smaller ATP symbols in the diagram, the cell makes a small amount of ATP during glycolysis and the citric acid cycle. The main function of these first two stages, however, is to supply the third stage of respiration with electrons (shown with gold arrows). Stage 3: Oxidative phosphorylation (purple background) involves electron transport and a process known as chemiosmosis. NADH and a related electron carrier, FADH2, shuttle electrons to electron transport chains embedded in the inner mitochondrial membrane. Most of the ATP produced by cellular respiration is generated by oxidative phosphorylation, which uses the energy released by redox reactions in the electron transport chain to make ATP. The electrons are finally passed to oxygen, which becomes reduced to H2O. What couples the electron transport chain to ATP synthesis? As electrons are passed down the energy staircase, the electron transport chain also pumps hydrogen ions (H+) across the inner mitochondrial membrane into the narrow intermembrane space (colored darker salmon in the figure). The result is a concentration gradient of H+ across the membrane. In chemiosmosis, the potential energy of this concentration gradient is used to make ATP. The term glycolysis (glyco, sweet, and lysis, split) means “splitting of sugar,” and that’s exactly what happens during this stage. Glycolysis begins with a single molecule of glucose and concludes with two molecules of pyruvate. Each represents a carbon atom in the molecules; glucose has six carbons, and these same six carbons end up in the two molecules of pyruvate (three carbons in each). The straight arrow running from glucose to pyruvate represents nine enzyme-catalyzed reactions. During these reactions, as glucose is oxidized, two molecules of NAD+ are reduced to two NADH, producing a net gain of two molecules of ATP. ATP is formed in glycolysis by substrate-level phosphorylation. In this process, an enzyme transfers a phosphate group from a substrate molecule to ADP, forming ATP. Later you will see that some ATP is also generated by substrate-level phosphorylation in the citric acid cycle (stage 2). However, the majority of ATP produced in cellular respiration involves an electron transport chain in stage 3. The breakdown of glucose to pyruvate releases energy, which is stored in ATP and NADH. The cell can use the energy in ATP immediately, but for it to use the energy in NADH, electrons from NADH must pass down an electron transport chain located in the inner mitochondrial membrane. The pyruvate molecules produced by glycolysis still possess about 90% of the energy available to the cell from the initial glucose molecule. In stage 2 of cellular respiration, pyruvate will be further oxidized, producing additional NADH to be used in stage 3. Before we move to stage 2, let’s take a closer look at glycolysis. The sequential steps of glycolysis illustrate how, in a metabolic pathway, each chemical step feeds into the next. In other words, the product of one reaction serves as the reactant for the next. Compounds that form between an initial reactant and a final product are known as intermediates. A specific enzyme catalyzes each chemical step (the figure does not include the enzymes). The steps of glycolysis can be grouped into two main phases. The energy investment phase consumes energy. In this phase, two molecules of ATP are used to add a phosphate group to each glucose molecule, which is then split into two small sugars. Steps 1–3 show how glucose is energized, using ATP. A sequence of three chemical reactions converts glucose to an energized intermediate. The curved arrows indicate the transfer of a phosphate group from ATP to another molecule. The cell invests 2 ATP, one at step 1 and one at step 3, to produce a more reactive molecule. In step 4, a six-carbon intermediate splits into two three-carbon intermediates. An enzyme splits the highly reactive six-carbon molecule into two three-carbon intermediates. Each of these molecules, called glyceraldehyde 3-phosphate (G3P), enters the next phase, so the steps in the energy payoff phase occur twice per glucose molecule. The steps in the energy payoff phase yield energy for the cell. This phase occurs after glucose has been split into two G3P molecules. Thus, the number 2 precedes all molecules in the diagram for this phase. In step 5, a redox reaction generates NADH. The curved arrow indicates the transfer of hydrogen atoms during a redox reaction as each G3P is oxidized and NAD+ is reduced to NADH. This reaction also attaches a phosphate group to the substrate. During steps 6–9, ATP and pyruvate are produced. This series of four chemical reactions completes glycolysis, producing two molecules of pyruvate for each initial molecule of glucose. In steps 6 and 9, ATP is produced by substrate-level phosphorylation, yielding a total of 4 ATP produced in the energy payoff phase. (Water is produced at step 8 as a by-product.) In sum, two NADH molecules are produced for each initial glucose molecule, and four total ATP are generated. Recall that the first phase used two molecules of ATP, so the net gain to the cell is two ATP molecules for each glucose that enters glycolysis. These two ATP molecules from glycolysis account for only about 6% of the energy that a cell can harvest from a glucose molecule. Some organisms—yeasts and certain bacteria, for instance—can satisfy their energy needs with the ATP produced by glycolysis alone. Some cells, such as your muscle cells, may use this anaerobic production of ATP for short periods when they do not have sufficient O2. Most cells and organisms, however, have far greater energy demands. The stages of cellular respiration that follow glycolysis release much more energy. In the next modules, we see what happens in most organisms after glucose is oxidized to pyruvate in glycolysis. As pyruvate is produced at the end of glycolysis, it is transported from the cytosol into a mitochondrion, where the citric acid cycle and oxidative phosphorylation occur. Recall that for each molecule of glucose that enters glycolysis, two molecules of pyruvate are produced. Each pyruvate enters a series of redox reactions that produce acetyl CoA and NADH. A large, multi-enzyme complex catalyzes these reactions in three steps. 1 Pyruvate loses a carbon and two oxygens as CO2. This is the first step in which CO2 is released during cellular respiration. 2 The remaining two-carbon compound is oxidized while a molecule of NAD+ is reduced to NADH. 3 A compound called coenzyme A joins with the two-carbon group to form a molecule called acetyl coenzyme A, abbreviated acetyl CoA. Acetyl CoA is then ready to enter the citric acid cycle. The citric acid cycle consists of a circular series of enzyme-catalyzed reactions. Several biochemical outputs are produced from one acetyl CoA: two molecules of CO2, one ATP molecule, three NADH molecules, and one molecule of the electron carrier FADH2. Remember that two acetyl CoA molecules were derived from two pyruvate molecules. Thus, the cycle runs twice, and the outputs are doubled for each glucose molecule processed. The ATP produced in the citric acid cycle can be used immediately by the cell, but most of the energy extracted from each glucose molecule is stored in NADH and FADH2. For the cell to be able to harvest the energy banked in NADH and FADH2, these molecules must shuttle their high-energy electrons to an electron transport chain (stage 3). Before we look at this last energy-producing stage of cellular respiration, we’ll take a detailed look at the steps of the citric acid cycle. There are six major steps of the citric acid cycle. 1 A turn of the citric acid cycle begins (top center) as enzymes strip the CoA portion from acetyl CoA and combine the remaining two-carbon group with the four-carbon molecule oxaloacetate (top left) already present in the mitochondrion. The product of this reaction is the six-carbon molecule, citrate (the ionized form of citric acid; hence the name citric acid cycle). In steps 2 and 3, NADH, ATP, and CO2 are generated during redox reactions. Successive redox reactions harvest energy by stripping hydrogen atoms from citrate and then alpha-ketoglutarate and producing reduced NADH. In two places, an intermediate compound loses a CO2 molecule. Energy is harvested by substrate-level phosphorylation of ADP to produce ATP. A four-carbon compound called succinate emerges at the end of step 3. Finally, in steps 4–6, further redox reactions generate FADH2 and more NADH. Succinate is oxidized as the electron carrier FAD is reduced to FADH2. Fumarate is converted to malate, which is then oxidized as one last NAD+ is reduced to NADH. One turn of the citric acid cycle is completed with the regeneration of oxaloacetate, which is ready to start the next cycle by accepting an acetyl group from acetyl CoA. Almost 90% of the ATP generated in cellular respiration is made in Stage 3, by oxidative phosphorylation, which involves electron transport and chemiosmosis. In this stage, we finally see how the electrons being carried by NADH and FADH2 from Stages 1 and 2 are used. Starting on the left in the illustration below, the gold arrows trace the transfer of electrons from NADH and FADH2 through the electron transport chain embedded in the inner membrane of the mitochondrion. The electron carriers in the chain sequentially pass electrons down the “energy staircase” to oxygen, the final electron acceptor. At three locations (green arrows), the energy released from these electron transfers enables the active transport of H+ into the intermembrane space. In the process called chemiosmosis, the resulting concentration gradient drives H+ through ATP synthase, an enzyme complex that phosphorylates ADP to ATP. Stage 3 is the big energy payoff that begins with a molecule of glucose. Glycolysis, which occurs in the cytosol, oxidizes glucose to two molecules of pyruvate, produces 2 NADH, and produces a net of 2 ATP by substrate-level phosphorylation. Within the mitochondrion, the oxidation of 2 pyruvate yields 2 NADH and 2 acetyl CoA. The 2 acetyl CoA feed into the citric acid cycle, which yields 6 NADH and 2 FADH2, as well as 2 ATP by substrate-level phosphorylation. Glucose has now been completely oxidized to CO2. NADH and FADH2 deliver electrons to the electron transport chain, where they are finally passed to O2, forming H2O. The energy released as electrons move down the “energy staircase” is used to pump H+ into the intermembrane space. The resulting H+ gradient is tapped by ATP synthase to produce about 28 molecules of ATP by oxidative phosphorylation. This capturing of energy from the oxidation of molecules via an electron transport chain used to phosphorylate ADP to ATP gives rise to the name oxidative phosphorylation. The total yield of ATP molecules per glucose is about 32. The number of ATP molecules cannot be stated exactly for several reasons. The NADH produced in glycolysis passes its electrons across the mitochondrial membrane to either NAD+ or FAD. Because FADH2 adds its electrons farther along the electron transport chain, it contributes less to the H+ gradient and thus generates less ATP. In addition, some of the energy of the H+ gradient may be used for work other than ATP production. Because most of the ATP generated results from oxidative phosphorylation, the ATP yield depends on an adequate supply of oxygen to the cell. Without oxygen to function as the final electron acceptor, electron transport and ATP production stop. But as we see next, some cells can oxidize organic fuel and generate ATP without oxygen. Fermentation is a way of harvesting energy that does not require oxygen. The pathway that generates ATP during fermentation is glycolysis, the same pathway that functions in the first stage of cellular respiration. Recall that glycolysis uses no oxygen; it simply generates a net gain of 2 ATP while oxidizing glucose to two molecules of pyruvate and reducing NAD+ to NADH. The yield of 2 ATP is certainly a lot less than the possible 32 ATP per glucose generated during aerobic respiration, but it is enough to keep your muscles contracting for a short time when oxygen is scarce. And many microorganisms supply all their energy needs through glycolysis. There is more to fermentation, however, than just glycolysis. To oxidize glucose in glycolysis, NAD+ must be present as an electron acceptor. This is no problem under aerobic conditions because the cell regenerates its pool of NAD+ when NADH passes its electrons into the mitochondrion to be transported to the electron transport chain. Fermentation provides an anaerobic path for recycling NADH back to NAD+. One common type of fermentation is called lactic acid fermentation. Your muscle cells and certain bacteria can regenerate NAD+ by this process. NADH is oxidized back to NAD+ as pyruvate is reduced to lactate. Muscle cells can switch to lactic acid fermentation when the need for ATP outpaces the delivery of O2 via the bloodstream. The lactate that builds up in muscle cells is thought to cause muscle soreness that occurs a day or so after intense exercise. Evidence shows, however, that within an hour, the lactate is carried by the blood to the liver, where it is converted back to pyruvate and oxidized. Muscle soreness is more likely caused by trauma to small muscle fibers, leading to inflammation and pain. For thousands of years, people have used alcohol fermentation in brewing, winemaking, and baking. Yeasts are single-celled fungi that normally use aerobic respiration to process their food. But they are also able to survive in anaerobic environments. Yeasts and certain bacteria recycle their NADH back to NAD+ while converting pyruvate to CO2 and ethanol. The CO2 provides the bubbles in beer and champagne. Bubbles of CO2 generated by baker’s yeast cause bread dough to rise. Ethanol, the two-carbon end product, is toxic to the organisms that produce it. Yeasts release their alcohol wastes to their surroundings, where it usually diffuses away. When yeasts are confined in a wine vat, they die when the alcohol concentration reaches 14%. Unlike muscle cells and yeasts, many prokaryotes that live in stagnant ponds and deep in the soil are called obligate anaerobes, meaning they require anaerobic conditions and are poisoned by oxygen. Yeasts and many other bacteria are called facultative anaerobes, and they can make ATP either by fermentation or by oxidative phosphorylation, depending on whether O2 is available. On the cellular level, our muscle cells behave as facultative anaerobes. For a facultative anaerobe, pyruvate is a fork in the metabolic road. If oxygen is available, the organism will always use the more productive aerobic respiration. Thus, to make wine and beer, yeasts must be grown anaerobically so that they will ferment sugars and produce ethanol. For this reason, the wine barrels and beer fermentation vats are designed to keep air out. Glycolysis is the universal energy-harvesting process of life. If you looked inside a bacterial cell, one of your body cells, or virtually any other living cell, you would find the metabolic machinery of glycolysis. The role of glycolysis in both fermentation and respiration has an evolutionary basis. Ancient prokaryotes are thought to have used glycolysis to make ATP long before oxygen was present in Earth’s atmosphere. The oldest-known fossils of bacteria date back more than 3.5 billion years, and they resemble some types of photosynthetic bacteria still found today. The evidence indicates, however, that significant levels of O2, formed as a by-product of bacterial photosynthesis, did not accumulate in the atmosphere until about 2.7 billion years ago. Thus, early prokaryotes most likely generated ATP exclusively from glycolysis, a process that does not require oxygen. The fact that glycolysis is the most widespread metabolic pathway found in Earth’s organisms today suggests that it evolved very early in the history of life. The location of glycolysis within the cell also implies great antiquity; the pathway does not require any of the membrane-enclosed organelles of the eukaryotic cell, which evolved about a billion years after the first prokaryotic cell. Glycolysis is a metabolic heirloom from early cells that continues to function in fermentation and as the first stage in the breakdown of organic molecules by cellular respiration. Throughout this chapter, we have spoken of glucose as the fuel for cellular respiration. But free glucose molecules are not common in your diet. You obtain most of your calories as carbohydrates (such as sucrose and other disaccharide sugars and starch, a polysaccharide), fats, and proteins. You consume all three of these classes of organic molecules when you eat a handful of peanuts, for instance. A wide range of carbohydrates can be funneled into glycolysis. For example, enzymes in your digestive tract hydrolyze starch to glucose, which is then broken down by cellular respiration. Similarly, glycogen, the polysaccharide stored in your liver and muscle cells, can be hydrolyzed to glucose to serve as fuel between meals. Fats make excellent cellular fuel because they contain many hydrogen atoms and thus many energy-rich electrons. As the diagram shows (tan arrows), a cell first hydrolyzes fats to glycerol and fatty acids. It then converts the glycerol to G3P, one of the intermediates in glycolysis. The fatty acids are broken into two-carbon fragments that enter the citric acid cycle as acetyl CoA. A gram of fat yields more than twice as much ATP as a gram of carbohydrate. Because so many calories are stockpiled in each gram of fat, you must expend a large amount of energy to burn fat stored in your body. This helps explain why it is so difficult for a dieter to lose excess fat. Proteins can also be used for fuel, although your body usually burns sugars and fats first. To be oxidized as fuel, proteins must first be digested to their constituent amino acids. Typically, a cell will use most of these amino acids to make its own proteins. Enzymes can convert excess amino acids to intermediates of glycolysis or the citric acid cycle, and their energy is then harvested by cellular respiration. During the conversion, the amino groups are stripped off and later disposed of in urine. Not all food molecules are destined to be oxidized as fuel for making ATP. Food also provides the raw materials your cells use for biosynthesis—the production of organic molecules using energy-requiring metabolic pathways. A cell must be able to make its own molecules to build its structures and perform its functions. Some raw materials, such as amino acids, can be incorporated directly into your macromolecules. However, your cells also need to make molecules that are not present in your food. Indeed, glycolysis and the citric acid cycle function as metabolic interchanges that enable your cells to convert some kinds of molecules to others as you need them. There are pathways by which your cells can make three classes of organic molecules using some of the intermediate molecules of glycolysis and the citric acid cycle. There are clear connections between the energy-harvesting pathways of cellular respiration and the biosynthetic pathways used to construct the organic molecules of the cell. The interconnections among these pathways provide a clear example of the theme of INTERACTIONS, in producing the emergent property of a balanced metabolism. Basic principles of supply and demand regulate these pathways. If there is an excess of a certain amino acid, for example, the pathway that synthesizes it is switched off. The most common mechanism for this control is feedback inhibition: The end product inhibits an enzyme that catalyzes an early step in the pathway. Feedback inhibition also controls cellular respiration. If ATP accumulates in a cell, it inhibits an early enzyme in glycolysis, slowing down respiration and conserving resources. On the other hand, the same enzyme is activated by a buildup of ADP in the cell, signaling the need for more energy. The cells of all living organisms—including those of the red panda and the plants it eats—have the ability to harvest energy from the breakdown of organic molecules. In the process of cellular respiration, the atoms of the starting materials end up in carbon dioxide and water. In contrast, the ability to make organic molecules from carbon dioxide and water is not universal. Animal cells lack this ability, but plant cells can produce organic molecules from inorganic ones using the energy of sunlight in the process of photosynthesis. Overall, Photosynthesis and cellular respiration provide energy for life. Photosynthesis uses solar energy to produce organic molecules and O2 from CO2 and H2O. In cellular respiration, O2 is consumed during the breakdown of organic molecules to CO2 and H2O, and energy is released; Breathing supplies O2 for use in cellular respiration and removes CO2; Cellular respiration banks energy in ATP molecules; The human body uses energy from ATP for all its activities; Cells capture energy from electrons “falling” from organic fuels to oxygen. Electrons removed from fuel molecules (oxidation) are transferred to NAD+ (reduction). NADH passes electrons to an electron transport chain. As electrons “fall” from carrier to carrier and finally to O2, energy is released; Cellular respiration occurs in three main stages. Stage 1: Glycolysis harvests chemical energy by oxidizing glucose to pyruvate. ATP is used to prime a glucose molecule, which is split in two. These three-carbon intermediates are oxidized to two molecules of pyruvate, yielding a net of 2 ATP and 2 NADH. ATP is formed by substrate-level phosphorylation, in which a phosphate group is transferred from an organic molecule to ADP; Multiple reactions in glycolysis split glucose into two molecules. Steps 1–4 consume energy, while steps 5–9 yield energy; The citric acid cycle completes the energy-yielding oxidation of organic molecules. The oxidation of pyruvate yields acetyl CoA, CO2, and NADH. For each turn of the citric acid cycle, two carbons from acetyl CoA are added, 2 CO2 are released, and 3 NADH and 1 FADH2 are produced; The multiple reactions of the citric acid cycle finish off the dismantling of glucose; Stage 3: Most ATP production occurs by oxidative phosphorylation. In mitochondria, electrons from NADH and FADH2 are passed down the electron transport chain to O2, which picks up H+ to form water. The energy released by these redox reactions is used to pump H+ into the intermembrane space. In chemiosmosis, the H+ gradient drives H+ back through ATP synthase complexes in the inner membrane, synthesizing ATP; Scientists have discovered heat-producing, calorie-burning brown fat in adults; Review: Each molecule of glucose yields many molecules of ATP. Up to 32 ATP molecules are produced for every glucose molecule oxidized in cellular respiration; Fermentation enables cells to produce ATP without oxygen. Under anaerobic conditions, muscle cells, yeasts, and certain bacteria produce ATP by glycolysis. NAD+ is recycled from NADH as pyruvate is reduced to lactate (lactic acid fermentation) or alcohol and CO2 (alcohol fermentation); Glycolysis evolved early in the history of life on Earth. Glycolysis occurs in the cytosol of the cells of nearly all organisms and is thought to have evolved in ancient prokaryotes; Cells use many kinds of organic molecules as fuel for cellular respiration; Organic molecules from food provide raw materials for biosynthesis. Cells use intermediates from cellular respiration and ATP for biosynthesis of other organic molecules. Metabolic pathways are often regulated by feedback inhibition.