Midterm 1 Study Guide PDF
Document Details
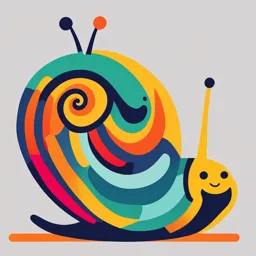
Uploaded by StainlessCosecant
Tufts University
Tags
Summary
This study guide provides definitions and explanations of common terminology related to the nervous system, including the central and peripheral nervous systems. It also summarizes key components of embryological development of the CNS and details specific structural formations. This guide is a good study resource for exams.
Full Transcript
Week 1 Define Common Terminology Used to Describe Specific Features within the Nervous System Term Definition Location...
Week 1 Define Common Terminology Used to Describe Specific Features within the Nervous System Term Definition Location Central nervous system Nucleus A group of neurons within the central nervous system (CNS) that share a similar function. (CNS). A group of neurons outside the central nervous system (CNS), essentially within the peripheral nervous Peripheral nervous Ganglia/Ganglion system (PNS), that share a similar function. system (PNS). Central nervous system Tract A bundle of axons within the central nervous system (CNS). (CNS). A bundle of axons outside the central nervous system (CNS), essentially within the peripheral nervous system Peripheral nervous Nerve (PNS). system (PNS). Afferent Information arriving in the body, generally sensory information. N/A Efferent Information exiting the body. N/A Brain and spinal cord Gray Matter Darker tissue of the brain and spinal cord, mainly composed of cell bodies. (CNS). Brain and spinal cord White Matter Paler tissue of the brain and spinal cord, mainly composed of axons. (CNS). Somatic Nervous Afferent and efferent information via skeletal muscles in the external environment, generally under conscious N/A System control. Autonomic Nervous Afferent and efferent information via smooth muscles and glands in the internal environment, generally N/A System automatic. Sulcus/Sulci Grooves of the brain. A large sulcus is called a fissure. Brain. Gyrus/Gyri Folds or ridges of the brain. Brain. Dorsal More superior aspect of the brain. The posterior aspect of the spinal cord. Brain and spinal cord. Ventral More inferior aspect of the brain. The anterior aspect of the spinal cord. Brain and spinal cord. Rostral More anterior towards the nose in the brain. More superior towards the brainstem in the spinal cord. Brain and spinal cord. More posterior towards the back of the head in the brain. More inferior towards the tail end of the spinal Caudal Brain and spinal cord. cord. Medial The portion of the brain in the midline. Brain. Lateral The portion of the brain that is further away from the midline. Brain. Horizontal Cut A transverse cut of the brain or spinal cord. Brain and spinal cord. Sagittal Cut A vertical cut of the brain or spinal cord. Brain and spinal cord. Coronal/Frontal Cut A vertical cut of the brain or spinal cord, from front to back. Brain and spinal cord. Central Nervous Consists of the brain and spinal cord. May be referred to as the upper motor neuron system. Protected by N/A System (CNS) bone. Peripheral Nervous Includes cranial nerves, peripheral nerves, anterior portion of the spinal cord, and cauda equina. May be N/A System (PNS) referred to as the lower motor neuron system. Central nervous Oligodendrocytes Glial cells that produce myelin in the central nervous system. system. Peripheral nervous Schwann cells Glial cells that produce myelin in the peripheral nervous system. system. Explain the Difference Between the Central and Peripheral Nervous System Feature Central Nervous System (CNS) Peripheral Nervous System (PNS) Cranial nerves, peripheral nerves, anterior portion of the spinal Components Brain and spinal cord cord, and cauda equina Other Names Upper motor neuron system Lower motor neuron system Protected by bone: skull/cranium (brain) and vertebral column (spinal Protection Not enclosed by bone cord) Neuron Groups Nuclei Ganglia or ganglion Axon Bundles Tracts Nerves Tissue Different tissue makeup, especially in the anterior portion of the spinal Gray matter (cell bodies) and white matter(axons) Composition cord Myelin Oligodendrocytes produce myelin Schwann cells produce myelin Production Resilience to More sensitive to change and injury, less resilient to healing N/A Injury Somatic Nervous Afferent and efferent information via skeletal muscles, generally under N/A System conscious control Autonomic Afferent and efferent information via smooth muscles and glands in the N/A Nervous System internal environment, generally automatic Function Processes and integrates information, generates responses. Transmits information between the CNS and the rest of the body. Medulla oblongata, pons, cerebellum, midbrain, thalamus, Examples Sciatic nerve, axillary nerve, cranial nerves, cauda equina hypothalamus, cerebral hemispheres and spinal cord Describe Key Components of Embryological Development of CNSFormation/Recall the Three Primary Vesicles and their eventual End- Structure Formation/Understand Key Time Points for Embryological Development Embryological Timeframe Key Events/Structures Outcomes/Significance Stage Formation of three layers: ectoderm, mesoderm, and endoderm. The ectoderm will Initial formation of the layers Roughly 18th differentiate into skin and nervous system including the brain and spinal cord. The that will give rise to the central Gastrulation day after mesoderm forms the notochord. The endoderm contributes to organ formation such as nervous system and other body conception respiratory and GI tracts. systems. Formation of the neural tube, the Neural plate forms from the ectoderm. The edges of the neural plate fold to form the neural precursor to the brain and spinal Roughly 3 to 4 groove and then fuse to create the neural tube. The inner layer of the neural tube will cord, and the differentiation of Neurulation weeks after become the gray matter and the outer layer will become the white matter. The neural gray and white matter, as well as gestation crest gives rise to ganglia of the nervous system. Somites form and will later develop into the development of structures that bone, muscle and dermis. form the sensory nerve roots of the spinal cord. The brain is initially divided into three main regions: the rhombencephalon (hindbrain), Starting the mesencephalon(midbrain), and the prosencephalon (forebrain). These regions continue Development of major brain around the to differentiate into other brain structures. The rhombencephalon develops into the structures and the ventricular Brain fourth week medulla oblongata, pons, and cerebellum, and parts of the fourth ventricle. The system. By the second trimester, Development of mesencephalon develops into the midbrain, and includes the cerebral aqueduct. The the brain begins to resemble an embryological prosencephalon develops into the thalamus and hypothalamus, portions of the lateral adult brain. development ventricles, and the cerebral hemispheres. Formation of the spinal cord and The neural tube forms the spinal cord. The alar componentsof somites will eventually its sensory and motor Spinal Cord Occurs during form the dorsal (sensory) nerve roots of the spinal cord, and the basal components will components. The spinal cord will Development neurulation form the ventral (muscle) nerve roots. The spinal cord grows slower than the vertebral not extend the full length of the column, and it stops growing at about L1 or L2 in adults. vertebral column. Explains the linear organization of Dermatome During Somite Somites start to split and change into the alar and basal components which influence the dermatomes in quadrupedal Development development layout of dermatomes position and how it appears in the traditional upright human. Essential for proper development Throughout Inductive Cells or tissues influence the fate of nearby cells via the secretion of chemical signals and differentiation of tissues and embryological Signaling (steroid-based or peptide-based hormones) structures within the nervous development system Review Different Neuronal Profiles within the Nervous System Neuron Type Description Location Function Multipolar Many dendrites and one axon extending from the cell body. This is Throughout the Transmits information within the CNS Neuron the most traditional form of a neuron. nervous system One axon and one dendrite extend from the soma in opposite Bipolar Neuron Retina of the eye Transmits sensory information directions. Pseudounipolar Transmits information from the periphery to the central nervous Transmits sensory information from the periphery Peripheral nerves Neuron system. to the central nervous system Understand the Differences Between Central and Peripheral Nerves Feature Central Nervous System (CNS) Peripheral Nervous System (PNS) Cranial nerves, peripheral nerves, anterior portion of the Components Brain and spinal cord spinal cord, and cauda equina Other Names Upper motor neuron system Lower motor neuron system Protected by bone: skull/cranium (brain) and vertebral column Protection Not enclosed by bone (spinal cord) Neuron Groups Nuclei Ganglia or ganglion Axon Bundles Tracts Nerves Tissue Different tissue makeup, especially in the anterior portion of the Gray matter (cell bodies) and white matter(axons) Composition spinal cord Myelin Oligodendrocytes produce myelin Schwann cells produce myelin Production Resilience to More sensitive to change and injury, less resilient to healing N/A Injury Somatic Afferent and efferent information via skeletal muscles, generally Nervous N/A under conscious control System Autonomic Afferent and efferent information via smooth muscles and glands Nervous N/A in the internal environment, generally automatic System Function Processes and integrates information, generates responses. Transmits information between the CNS and the rest of the body. Medulla oblongata, pons, cerebellum, midbrain, thalamus, Examples Sciatic nerve, axillary nerve, cranial nerves, cauda equina hypothalamus, cerebral hemispheres and spinal cord The central nervous system (CNS) and peripheral nervous system (PNS) are the two main components of the nervous system, each with distinct structures and functions. Central Nervous System (CNS): The CNS consists of the brain and spinal cord. It is also referred to as the upper motor neuron system. The CNS is protected by bone: the skull or cranium surrounds the brain and the vertebral column protects the spinal cord. Within the CNS, groups of neurons are called nuclei, and bundles of axons are called tracts. The CNS is made up of gray matter, which consists mainly of cell bodies, and white matter, which consists mainly of axons. Oligodendrocytes are the glial cells that produce myelin within the CNS. The CNS is more sensitive to change and injury and less resilient to healing. Examples of structures include the medulla oblongata, pons, cerebellum, midbrain, thalamus, hypothalamus, cerebral hemispheres and spinal cord. Peripheral Nervous System (PNS): The PNS includes the cranial nerves, peripheral nerves, the anterior portion of the spinal cord, and the cauda equina. It is also referred to as the lower motor neuron system. The PNS is not enclosed by bone. Groups of neurons within the PNS are called ganglia or ganglion, and bundles of axons are called nerves. The PNS has a different tissue makeup, especially in the anterior portion of the spinal cord. Schwann cells are the glial cells that produce myelin in the PNS. The PNS contains the somatic nervous system, which controls afferent and efferent information via skeletal muscles, and the autonomic nervous system which controls afferent and efferent information via smooth muscles and glands. Examples of peripheral nerves are the sciatic nerve and the axillary nerve. In summary, the CNS is the control center of the nervous system, processing and integrating information, while the PNS transmits information between the CNS and the rest of the body. The CNS is protected by bone, contains nuclei and tracts, and has gray and white matter. The PNS is not enclosed by Review the Basic Ions and Channels During Action Potential Ions Sodium (Na+), chloride (Cl-), and calcium (Ca2+) ions are typically found in higher concentrations outside the neuron and tend to move into the neuron based on their concentration gradients. Potassium (K+) is more concentrated inside the neuron and tends to move outside the neuron. The movement of these ions across the membrane generates an electrical impulse. Ion Channels Ligand-gated ion channels open in response to specific neurotransmitters binding to them. This is like a key fitting into a lock. Voltage-gated channels open in response to changes in electrical potential across the membrane. Modality-gated channels open in response to mechanical forces such as the stretch of a muscle or changes in temperature. Ion pumps help to establish and maintain the concentration gradient of ions inside and outside the cell. They set the stage for the movement of ions needed for an action potential. Review the Mechanism of a Nerve Action Potential An action potential is a rapid change in the electrical potential of a neuron's membrane, allowing for nerve signal transmission. It involves a sequence of events related to the movement of ions across the membrane through various channels and pumps. Here is a review of the mechanism of a nerve action potential: Resting Membrane Potential: A neuron at rest has a negative charge and there is no net movement of ions across the membrane. The membrane is somewhat "leaky", but the small movement of ions is not enough to cause a significant change. Local Potential: When a neuron receives a stimulus, a few ions begin to move across the membrane, creating a small change in the membrane potential called a local potential. This change is not always enough to trigger an action potential. Depolarization and Action Potential: If the stimulus is strong enough, there is a large influx of ions across the membrane, leading to an action potential. This is an all-or-nothing event that is actively propagated along the axon. ◦ Voltage-gated sodium channels open, allowing a large influx of sodium (Na+) into the neuron. This causes the membrane potential to become positive. ◦ As sodium rushes in, potassium (K+) starts to leak out of the membrane. Repolarization: The sodium channels become inactive, and potassium continues to move out of the cell, helping to restore the negative resting membrane potential. This process is called repolarization. Hyperpolarization: There is a brief period of hyperpolarization, where the membrane potential becomes even more negative than at rest. During this time, the neuron is unable to fire another action potential. Return to Resting Potential: The sodium-potassium pumps restore the ion concentrations to their resting state, and the membrane potential returns to its baseline negative charge. Propagation of the Action Potential: The action potential propagates down the axon via a chain reaction. ◦ Sodium rushes in, causing a depolarization. ◦ Potassium leaks out behind the depolarization. ◦ This process continues down the axon. ◦ The refractory period created by the potassium outflow prevents the action potential from traveling backwards. Saltatory Conduction: In myelinated axons, the action potential "jumps" from one node of Ranvier to the next in a process called saltatory conduction, allowing for faster transmission of the signal. The action potential will travel down the axon to the presynaptic terminal to then communicate with a neighboring neuron In summary, the action potential is a complex sequence of events involving the movement of ions like sodium and potassium across the neuron's membrane. This process is critical for nerve communication and signal transmission. Explain the Temporal Sequence of Nerve Conduction The temporal sequence of nerve conduction involves a series of events that facilitate the transmission of electrical signals along a neuron, leading to communication with other neurons or target cells. The process can be broken down into the following steps: Resting Membrane Potential: Initially, a neuron is at rest with a negative charge, and there is no net movement of ions across the membrane. Although the membrane is somewhat leaky, the movement of individual ions is not significant enough to cause a major change. This is the baseline state before any signal transmission occurs. Stimulus Reception: A neuron receives a stimulus, which could be a chemical signal from a neighboring neuron, or some other stimulus. Local Potential: In response to a stimulus, a few ions start to move across the neuronal membrane, causing a small change in the membrane potential. This is called a local potential, and is not enough to generate an action potential. Depolarization and Action Potential Initiation: If the local potential reaches a certain threshold, it triggers a large and rapid change in membrane potential, known as the action potential. This is an all-or-nothing event. ◦ Voltage-gated sodium channels open, allowing a massive influx of sodium ions (Na+) into the neuron. This causes the membrane potential to become positive. ◦ As sodium floods in, potassium ions (K+) start to leak out of the membrane. Propagation of the Action Potential: The action potential then travels down the axon via a domino effect. ◦ The influx of sodium at one point on the axon triggers depolarization in the adjacent area. ◦ The outflow of potassium behind the depolarization creates a refractory period, which prevents the action potential from traveling backwards. Repolarization: After depolarization, the sodium channels become inactive, and potassium continues to move out of the cell. This outward flow of potassium restores the negative resting membrane potential in a process known as repolarization. Hyperpolarization: There is a brief period of hyperpolarization where the membrane potential becomes even more negative than at rest. During this time the neuron is unable to fire another action potential. Return to Resting Potential: The sodium-potassium pumps work to restore the ion concentrations to their resting state, bringing the membrane potential back to its baseline negative charge. Saltatory Conduction: In myelinated axons, the action potential jumps from one node of Ranvier to the next in a process called saltatory conduction, which increases the speed of signal transmission. Synaptic Transmission: The action potential reaches the presynaptic terminal at the end of the axon. This triggers the release of neurotransmitters into the synaptic cleft, which is the space between neurons. Postsynaptic Potential: Neurotransmitters bind to receptors on the postsynaptic neuron and cause an electrical signal called the postsynaptic potential in that receiving neuron. The entire process starts again in the postsynaptic neuron to propagate the signal further. In summary, nerve conduction is a sequence of electrochemical events starting with a stimulus, progressing through depolarization and repolarization, and finally culminating in synaptic transmission to the next neuron. This precise sequence is essential for rapid and reliable communication within the nervous system. Review Common Neuro Transmitters Neurotransmitters are signaling molecules that facilitate communication between neurons. They are released from the presynaptic terminal, travel across the synaptic cleft, and bind to postsynaptic receptors on a neighboring neuron. There are two classes of neurotransmitters: small molecules and peptides. Here are some common neurotransmitters: Acetylcholine is a major neurotransmitter in the peripheral nervous system, and is important for muscle contraction. Glutamate is a major neurotransmitter in the central nervous system and is used in almost every part of the brain. GABA is a major inhibitory neurotransmitter in the central nervous system. It can slow down nervous system transmission and is used in treatments for anxiety and seizures. Catecholamines can be excitatory or inhibitory. The sources do not provide further information on the specific types or functions of catecholamines. Excitatory vs. Inhibitory Neurotransmitters All neurons projecting to the white matter are excitatory in nature. Excitatory neurotransmitters, like acetylcholine and glutamate, promote the transmission of signals. Inhibitory neurotransmitters, like GABA, slow down or prevent the transmission of signals. Other important information about neurotransmitters Endocannabinoids are released by the postsynaptic membrane in response to a strong excitation. They move retrogradely across the synapse and bind to presynaptic terminals, causing an inhibitory effect by limiting the amount of neurotransmitter released. Neurotransmitters require calcium to get to the synaptic cleft and proceed to the postsynaptic receptors. After release into the synaptic cleft, neurotransmitters can bind to ionotropic receptors or metabotropic receptorson the postsynaptic membrane. ◦ Ionotropic receptors are ion channels that open or close in response to the binding of a neurotransmitter and are very fast. ◦ Metabotropic receptors do not form ion channels directly, and use transduction mechanisms that are slower. Pharmacological Relevance Botox limits the amount of calcium present in the presynaptic terminal which limits the amount of acetylcholine released, causing paralysis. Myasthenia gravis is an autoimmune disease that destroys postsynaptic receptors, which does not allow the muscle to contract because the message cannot get through, leading to weakness. In summary, neurotransmitters are crucial for nerve communication. They can be excitatory or inhibitory and are involved in many different aspects of nervous system function. Explain the Difference Between Electrical vs. Chemical Synapse Electrical and chemical synapses are two distinct mechanisms by which neurons communicate with each other, each with its own unique characteristics. Electrical Synapses: Mechanism: Electrical synapses use gap junctions that directly connect the cytoplasm of two neurons, allowing ions to flow directly from one cell to another. This direct flow of ions enables very rapid communication. Speed: Communication at electrical synapses is very fast due to the direct passage of ions. Prevalence: Electrical synapses are less common in humans and are found in a few neurons in the spinal cord and the hippocampus. Signal Transmission: Signal transmission is primarily electrical, involving the direct flow of ions through gap junctions. Role of Calcium: The sources do not explicitly state that calcium is required for signal transmission in electrical synapses. Neurotransmitters: Neurotransmitters are not used in electrical synapses. Postsynaptic Receptors: Postsynaptic receptors are not applicable in electrical synapses, as signals are transmitted directly through gap junctions. Signal Type: The signal is primarily electrical. Chemical Synapses: Mechanism: Chemical synapses use neurotransmitters to transmit signals across the synaptic cleft, which is the space between neurons. Speed: Communication at chemical synapses is slower compared to electrical synapses, as it involves several steps, including the release of neurotransmitters, diffusion across the synaptic cleft, and binding to postsynaptic receptors. Prevalence: Chemical synapses are the more common type of synapse, with many found throughout the nervous system. Signal Transmission: Signal transmission is primarily chemical, involving the release of neurotransmitters that diffuse across the synaptic cleft to bind to postsynaptic receptors. Role of Calcium: An influx of calcium into the presynaptic terminal is essential to facilitate the release of neurotransmitters. Neurotransmitters: Neurotransmitters are required for signal transmission. They are released from the presynaptic terminal, travel across the synaptic cleft and bind to the postsynaptic receptors. Postsynaptic Receptors: Neurotransmitters bind to ionotropic or metabotropic receptors on the postsynaptic membrane, continuing the signal. ◦ Ionotropic receptors are ion channels that open or close in response to the binding of a neurotransmitter and are very fast. ◦ Metabotropic receptors do not form ion channels directly, and use transduction mechanisms that are slower. Signal Type: The signal is primarily chemical but includes a postsynaptic electrical signal dependent on the chemical transmission across the synaptic cleft. In summary, electrical synapses allow for direct and rapid communication via gap junctions, while chemical synapses use neurotransmitters for slower but more versatile communication across the synaptic cleft. Understand the Different Post-Synaptic Receptors Postsynaptic receptors are located on the dendrites of a neuron and are essential for receiving and processing signals transmitted from a presynaptic neuron. There are two main types of postsynaptic receptors: ionotropic and metabotropic. Ionotropic Receptors Mechanism: Ionotropic receptors are ion channels that open or close in response to the binding of a neurotransmitter. They are ligand-gated ion channels, meaning they are very selective about which ions they allow to pass through. Speed: They facilitate very fast transmission across the membrane. Function: They allow for quick changes in membrane potential by allowing ions to flow into the postsynaptic neuron. Metabotropic Receptors Mechanism: Metabotropic receptors do not form an ion channel pore. Instead, they use transduction mechanisms to initiate a series of events within the postsynaptic neuron. Speed: They are slower compared to ionotropic receptors due to the additional steps involved in their signaling process. Function: They can trigger a variety of intracellular responses, such as changes in gene expression, or metabolic activity in the postsynaptic cell. Examples: Examples of metabotropic receptors include glutamate receptors, muscarinic or acetylcholine receptors, and GABA receptors. Key Differences Summarized Speed: Ionotropic receptors are fast, while metabotropic receptors are slower. Mechanism: Ionotropic receptors are ion channels, whereas metabotropic receptors use transduction mechanisms. Function: Ionotropic receptors directly alter membrane permeability to ions, while metabotropic receptors trigger intracellular changes, that can lead to a variety of responses in the postsynaptic cell. Both types of receptors play a critical role in synaptic transmission by facilitating the process of transforming a chemical signal (neurotransmitter) into an electrical signal in the postsynaptic neuron. Understand the Different Neuroglia and Their Contribution to Neuronal Function Neuroglia, also known as glial cells, are non-neuronal cells in the nervous system that support neurons and their functions. These cells play a critical role in maintaining the health and functionality of the nervous system. They support metabolic processes and signaling functions of neurons, participate in neuron circuit formation, and contribute to synaptic plasticity. Glial cells also help make myelin, contribute to the blood- brain barrier, and participate in inflammatory responses. There are several types of glial cells, each with specific roles. The glial cells found within the central nervous system (CNS) include astrocytes, microglia, and oligodendrocytes. Astrocytes Astrocytes are typically found in the gray matter of the CNS. They are called "star cells" because of their star-like shape. Astrocytes maintain the ionic balance in the extracellular fluid and regulate the concentration of ions like calcium. They also act as a "waste management" system by taking up and processing neurotransmitters released in the synaptic cleft. Tumors such as astrocytomas or glioblastomas can originate from astrocytes. Microglia Microglia are derived from bone marrow and are found in the CNS. They act as phagocytes, clearing debris and modulating inflammatory processes within the CNS. In neurodegenerative diseases, microglia may not function properly and may contribute to excess debris. Oligodendrocytes Oligodendrocytes are located in the CNS and are responsible for producing myelin. They produce multiple layers of myelin at a time to insulate axons and help facilitate nerve transmission. Myelin and its importance Myelin is a fatty protein that surrounds the axon of a nerve to insulate it. It allows for fast and efficient transduction of electrical signals. Myelin is not continuous but is segmented with gaps called nodes of Ranvier. The nodes of Ranvier contain high densities of voltage-gated channels, allowing for faster current spread. Saltatory conduction occurs in myelinated fibers, where the action potential "jumps" from one node of Ranvier to the next, facilitating faster signal transmission. Oligodendrocytes produce myelin in the CNS. Comparison of Glial Cells in the CNS and PNS Feature CNS Glial Cells PNS Glial Cells Types Astrocytes, Microglia, Oligodendrocytes Satellite cells, Schwann cells Myelin Production Oligodendrocytes Schwann cells Layers of Myelin Multiple layers at a time Fewer layers, one or two at a time Clinical relevance of glial cells Multiple sclerosis (MS) is an autoimmune disease where myelin in the CNS is stripped off, leading to various symptoms like muscle tone changes, weakness, and sensory deficits. Guillain-Barré syndrome is an autoimmune disease where myelin in the peripheral nervous system is stripped off, leading to muscle weakness, atrophy, and sensory deficits. In summary, neuroglia play a vital role in supporting neurons and their functions. They maintain the neuronal environment, help transmit signals, and contribute to the overall health and functionality of the nervous system. Describe the Difference Between a Central and Peripheral System Demyelinating Event Demyelinating events occur when the myelin sheath, which insulates and protects nerve fibers, is damaged or destroyed. This can happen in both the central nervous system nervous system (PNS), leading to different clinical manifestations due to the distinct characteristics of these two systems. Here are key differences between central and peripheral demyelinating events: Central Nervous System (CNS) Demyelination Location: Demyelination occurs within the brain and spinal cord. Glial Cells Involved: Oligodendrocytes are the glial cells responsible for producing myelin in the CNS. Disease Example: A primary example of a CNS demyelinating disease is multiple sclerosis (MS). Cause: MS is an autoimmune disease where the body's immune system attacks and damages the myelin sheaths in the CNS. Genetic and environmental factors ma disease. Symptoms: Symptoms of MS can vary but typically include: ◦ Increased muscle tone or spasticity ◦ Muscle weakness ◦ Hyper-reflexive reflexes ◦ Sensory deficits such as numbness and tingling Prognosis and Treatment: The prognosis of MS is variable and depends on the stage of the disease. Treatment includes disease-modifying drugs to slow progressio rehabilitative services. Peripheral Nervous System (PNS) Demyelination Location: Demyelination occurs in the nerves outside the brain and spinal cord. Glial Cells Involved: Schwann cells are the glial cells responsible for producing myelin in the PNS. They produce less myelin and do it in stages. Disease Example: A primary example of a PNS demyelinating disease is Guillain-Barré syndrome. Cause: Guillain-Barré syndrome is thought to be triggered by a viral or bacterial infection, leading to an autoimmune response where the body attacks myelin in the Symptoms: Symptoms of Guillain-Barré syndrome include: ◦ Muscle weakness ◦ Muscle atrophy (shrinkage) ◦ Diminished or absent reflexes ◦ Sensory deficits Prognosis and Treatment: The prognosis for Guillain-Barré syndrome is generally more favorable than for MS, with a higher likelihood of recovery. Treatment inv immunosuppressant drugs like IVIG. Key Differences Summarized: Feature CNS Demyelination (e.g., MS) PNS Demyelination (e.g., Guillain-Barré) Location Brain and spinal cord Nerves outside the brain and spinal cord Glial Cells Oligodendrocytes Schwann cells Primary Disease Multiple Sclerosis (MS) Guillain-Barré syndrome Cause Autoimmune, genetic, environmental Viral or bacterial infection triggering autoimmune response Muscle Symptoms Spasticity, weakness Weakness, atrophy Reflexes Hyper-reflexive Diminished or absent Sensory Deficits Numbness, tingling Sensory deficits Prognosis Variable, often progressive More recoverable Treatment Disease-modifying drugs, rehab Immunosuppressants, supportive care Impact of Demyelination on Nerve Transmission Myelin's Role: Myelin is a fatty protein that insulates axons, allowing for the fast and efficient conduction of electrical signals. Saltatory Conduction: In myelinated axons, action potentials "jump" from one node of Ranvier to the next, a process known as saltatory conduction, speeding up tr Effect of Demyelination: When myelin is damaged, the transmission of nerve signals is slowed or blocked, leading to the symptoms associated with demyelinating In summary, while both central and peripheral demyelinating events involve the destruction of myelin, they differ in their location, the glial cells involved, the specific disea symptoms. The clinical manifestations of demyelination are a consequence of slowed or blocked nerve signal transmission. Guiding Questions Can you describe the general flow of information of how a nerve transmits information? 2. How is an action potential generated and what are the major events? 3. Can you describe the steps with how a neuron communicates with it’s neighboring neuron at the presynaptic terminal? 4. How does myelin assist with nerve conduction? 1. What are the consequences to a lack of myelination? Here's a breakdown of how nerves transmit information, action potential generation, synaptic communication, myelin's role, and the consequences of demyelination based on the sources and our conversation history: 1. General Flow of Nerve Information: A neuron receives information through its dendrites and soma (cell body). The information is then conducted through the axon. Finally, the information is transmitted to the next neuron through the presynaptic terminal. Generally, a nerve transmits information in one direction, from the dendrites to the presynaptic terminal. 2. Action Potential Generation and Major Events: Ions such as sodium, chloride, and calcium want to move into the neuron, while potassium wants to move out, based on their concentrations. Ion channels, including ligand-gated, voltage-gated, and modality-gated channels, control the movement of ions. Ion pumps help establish the ion concentration gradient. Resting Potential: A neuron at rest is negatively charged, with no net flow of ions across the membrane. Local Potential: A small ionic flow occurs across the membrane, not enough to generate an impulse. Action Potential: When there's a large enough ionic flow, an action potential is generated which is an "all-or-nothing" event. ◦ Depolarization: Voltage-gated sodium channels open, causing a massive influx of sodium into the cell. ◦ Repolarization: Potassium channels open, allowing potassium to flow out of the cell, restoring the resting membrane potential. ◦ Hyperpolarization: A brief period where the neuron is even more negative than at rest, making it unable to fire. The action potential is actively propagated along the axon. 3. Steps of Neuronal Communication at the Presynaptic Terminal: Calcium Influx: Calcium ions enter the presynaptic terminal through the membrane. Neurotransmitter Release: Calcium ions bind to proteins that contain vesicles of neurotransmitters, which then release the neurotransmitters into the synaptic cleft. Neurotransmitter Binding: The neurotransmitter travels across the synaptic cleft to bind to postsynaptic receptors on the dendrites of the neighboring neuron. Postsynaptic Effects: The binding of neurotransmitters to receptors will either excite or inhibit the postsynaptic neuron, influencing the potential for the postsynaptic neuron to fire its own action potential. There can be either chemical or electrical synapses. Chemical synapses use neurotransmitters to communicate across the synaptic cleft. Electrical synapses use gap junctions for rapid communication. 4. Myelin's Assistance with Nerve Conduction: Myelin is a fatty protein that insulates the axon, allowing for faster and more efficient signal transduction. Myelin is not continuous but is segmented, with gaps called nodes of Ranvier. Nodes of Ranvier have high densities of voltage-gated channels, allowing for faster current spread. Saltatory conduction is the process where the action potential "jumps" from one node of Ranvier to the next, facilitating faster signal transmission. 5. Consequences of Lack of Myelination (Demyelination): Slowed or Blocked Transmission: When myelin is damaged, the transmission of nerve signals is slowed or blocked. Central Nervous System Demyelination (e.g., Multiple Sclerosis): ◦ Occurs in the brain and spinal cord. ◦ Involves oligodendrocytes. ◦ Results in symptoms like increased muscle tone, weakness, hyper-reflexia, and sensory deficits. Peripheral Nervous System Demyelination (e.g., Guillain-Barré Syndrome): ◦ Occurs in the nerves outside the brain and spinal cord. ◦ Involves Schwann cells. ◦ Results in symptoms like muscle weakness, atrophy, diminished reflexes and sensory deficits. In summary, the nervous system uses a combination of electrical and chemical signals to transmit information. Myelin plays a critical role in speeding up this process. When demyelination occurs, it disrupts the transmission of nerve signals and causes various neurological issues. Week 2 Cerebral Topography- Surface Features (landmarks)/Review Key Landmark Sulci and Guryi and Identify Related Brain Structures/Identify and describe lobes of the cerebral cortex—Frontal, Temporal, Parietal, Occipital, and Insular Key landmarks and surface features of the cerebrum and cerebral cortex include: Cerebrum: The most significant part of the brain, responsible for higher functions such as interpreting touch, vision, hearing, speech, reasoning, emotions, learning, and movement. It contains two cerebral hemispheres and several subcortical structures. Cerebral Cortex: The outermost layer of neural tissue (gray matter) covering the cerebrum. It is extensively folded into ridges (gyri) and grooves (sulci). If flattened, it would be about one square meter, but the foldings allow this large surface area to fit within the skull. The cerebral cortex is divided into motor, sensory, and association areas. ◦ Gyri: Ridges on the surface of the cerebral cortex. ◦ Sulci: Grooves or depressions in the cerebral cortex that separate the gyri. ◦ Longitudinal Fissure (Sagittal Fissure): The deepest groove that separates the two cerebral hemispheres. It runs along the midline of the cortex. ◦ Transverse Cerebral Fissure: Separates the cerebrum from the cerebellum. Lobes: The cerebral hemispheres are divided into four lobes: ◦ Frontal Lobe: Lies anterior to the central sulcus and is the largest lobe. It is the control panel for behavior and complex thinking abilities, managing thinking, emotions, personality, judgment, self-control, muscle control, movements, and memory storage. Substructures of the frontal lobe include the prefrontal cortex, the orbital frontal cortex, the motor cortex, the premotor cortex, and Broca's area. ◦ Parietal Lobe: Lies posterior to the central sulcus, and is located at the top rear of the head. It processes and integrates sensory information like touch, temperature, pressure, and pain. It also plays a role in spatial orientation and body position (proprioception). ◦ Occipital Lobe: Located at the back of the head, separated from the parietal lobe by the parietal-occipital sulcus. It is the visual processing hub of the brain and contains the primary visual cortex, which receives visual information from the eyes. ◦ Temporal Lobe: Located behind the ears. They are associated with auditory processing, understanding language, memory formation, and emotion regulation. ◦ Insular Lobe (Insular Cortex): Folded between the frontal, parietal, and temporal lobes, deep within the lateral sulcus. It is a major hub in emotional regulation, memory, multi-sensory integration, and autonomic control. Key Sulci: ◦ Central Sulcus: Delineates the frontal lobe from the parietal lobe. ◦ Lateral Sulcus: Divides the hemispheres into the frontal, parietal, temporal and occipital lobes. ◦ Parieto-occipital Sulcus: Separates the parietal lobe from the occipital lobe. ◦ Calcarine Sulcus: Borders the cuneus, a region of the occipital lobe involved in visual processing. Key Gyri: ◦ Precentral Gyrus: Located in the frontal lobe, entirely motor in function. The primary motor cortex is located here and is responsible for voluntary motor control. ◦ Postcentral Gyrus: Located in the parietal lobe, entirely sensory in function. The primary somatosensory cortex is located here. ◦ Cingulate Gyrus: Part of the limbic system, involved in emotion, behavior, pain, memory, attention, and autonomic motor function. ◦ Superior Frontal Gyrus, Middle Frontal Gyrus, Inferior Frontal Gyrus: Landmarks on the frontal lobe. ◦ Superior Temporal Gyrus, Middle Temporal Gyrus, Inferior Temporal Gyrus: Landmarks on the temporal lobe. Other Landmarks and Structures: ◦ Corpus Callosum: A massive band of white matter connecting the cortex of the two hemispheres. It includes the rostrum, genu, body, and splenium. ◦ Anterior Commissure: Lies below the rostrum of the corpus callosum. ◦ Olfactory Bulbs: Rounded masses of tissue in the brain that process information about smells. ◦ Optic Chiasm: An X-shaped structure where optic nerve fibers from each eye cross over, allowing for the occipital cortex to process vision from both eyes. ◦ Thalamus: An egg-shaped structure in the middle of the brain that acts as a relay station for incoming motor and sensory information. ◦ Cerebellum: Located under the cerebrum, coordinates muscle movements, and maintains posture and balance. ◦ Brainstem: A relay center connecting the cerebrum and cerebellum to the spinal cord. Includes the midbrain. ◦ Uncus: A hook-shaped structure in the temporal lobe that extends medially towards the midbrain, involved in memory, emotions and smell. ◦ Cuneus: A wedge-shaped region of the brain's occipital lobe that plays a role in visual processing. ◦ Limbic Lobe: A C-shaped compilation of brain structures that are involved with memory, emotion, learning, motivation and other functions as it has a touch on the frontal, parietal and temporal lobes. These landmarks and features are essential for understanding the organization and function of the brain. Understand and Integrate the Cortical Homunculus to Pair with Specific Regions of the Brain The cortical homunculus is a visual representation of the body within the brain, specifically within the primary motor and somatosensory cortices. It illustrates how differen parts of the body are mapped onto these areas of the brain. The homunculus helps to understand which parts of the brain are responsible for controlling specific body parts. T concept is crucial for diagnosing and treating neurologic conditions. Here's how the cortical homunculus relates to specific regions of the brain: Primary Motor Cortex: ◦ This area is located in the precentral gyrus of the frontal lobe. ◦ The motor homunculus shows which regions of the motor cortex correspond to movements of different body parts. ◦ Body parts requiring fine motor control, such as the hands and face, are represented by larger areas on the motor homunculus. ◦ The primary motor cortex is responsible for voluntary motor control. ◦ The precentral gyrus, where the primary motor cortex is, is anterior to the central sulcus Primary Somatosensory Cortex: ◦ This area is located in the postcentral gyrus of the parietal lobe. ◦ The sensory homunculus maps out which regions of the somatosensory cortex correspond to sensations from different body parts. ◦ Body parts with more sensory receptors, such as the lips and fingertips, are represented by larger areas on the sensory homunculus. ◦ The primary somatosensory cortex processes sensory information from the body, such as touch, temperature, and pain. ◦ The postcentral gyrus, where the primary somatosensory cortex is, is posterior to the central sulcus. Key Features of Both Homunculi: ◦ Distorted Proportions: Both homunculi are characterized by exaggerated features. For example, the hands, lips, and face appear disproportionately large compared to other body parts. This reflects the density of nerve endings and the complexity of movements or sensations in those areas. ◦ Contralateral Representation: Each hemisphere of the brain controls and receives information from the opposite side of the body. The left hemisphere's homunculus represents the right side of the body, and vice versa. Clinical Significance: ◦ Understanding the homunculus is important for physical therapists when designing targeted rehab programs for patients recovering from strokes or injuries, b focusing on the affected areas of the homunculus. ◦ The homunculus helps to understand how the brain dedicates different amounts of cortical space to various body parts based on their sensory and motor dema Association Areas: Each lobe of the brain has association areas that integrate information from multiple brain regions. This adds complexity to sensory perceptions o facilitates higher cognitive or motor processes. The cortical homunculus is a fundamental concept in neuroscience that enhances the understanding of brain function, guides clinical practices, and informs research and education. It is also important in pairing brain regions with vascular supply, as the location of a stroke will dictate the symptoms experienced due to the areas of the homuncu and corresponding regions of the brain affected by the stroke. Identify and Explain the Circle of Willis/Identify and Describe the Main Arterial Vasculature of the Brain/Identify and Describe Aspects of the Brain’s Venous System The Circle of Willis is a ring-shaped arterial formation at the base of the brain that connects the internal carotid arteries to the vertebral arteries. This structure is located in the subarachnoid space and surrounds the pituitary gland and optic chiasm. The main function of the Circle of Willis is to provide collateral circulation to the brain. This means that if one of the major arteries supplying the brain becomes blocked, blood can still flow to that area through the other arteries in the circle. This is important because it can help to reduce the severity of a stroke. The main components of the Circle of Willis are: Right and left anterior cerebral arteries Anterior communicating artery Right and left internal carotid arteries Right and left posterior cerebral arteries Right and left posterior communicating arteries Basilar artery Vertebral arteries (which bifurcate off the basilar artery) The internal carotid arteries and vertebral arteries are the two primary blood flow pathways to the brain. The internal carotid arteries supply the anterior two-thirds of the cerebral hemispheres. This includes the deep white matter and the basal ganglia. The vertebral and basilar arteries supply the remaining posterior and medial regions of the cerebral hemispheres, the brainstem, cerebellum, and cervical spinal cord. The anterior and posterior communicating arteries connect the anterior and posterior circulations of the brain. These small arteries are important because they can provide alternate routes for blood flow if one of the larger arteries is blocked. It is important to note that not everyone has a complete Circle of Willis. Studies show that 50-90% of people have some variation in the anatomy of their Circle of Willis. These variations can affect the risk and impact of cerebrovascular events, such as strokes. Understanding the Circle of Willis is essential for interpreting imaging studies and understanding patients with neurological conditions. This knowledge helps therapists assess and understand the underlying causes of a patient’s symptoms and can assist in designing effective rehabilitation programs. Artery Supplies Damage Contralateral hemiplegia (more in the leg than the arm) Sensory loss (especially in the foot and lower Anterior Medial portions of the frontal lobes extremity) Impaired consciousness Apathy and indifference to stimuli Loss of executive functions Cerebral and superior medial parietal lobes Frontal lobe behavioral abnormalities Speech dysfunctions (e.g., aphasia) Apraxia Urinary Artery (ACA) incontinence Contralateral hemiplegia (more in the arm and face than the leg) Sensory loss, including vision, Middle Large portion of the lateral cerebral hearing, smell, taste, and touch Aphasia (if in the dominant hemisphere) or dysarthria Swallowing Cerebral cortex, including the frontal, issues Hemineglect or inattention (if in the non-dominant hemisphere) Vision deficits or homonymous Artery (MCA) temporal, and parietal lobes hemianopsia Occipital lobe, inferior part of the Posterior temporal lobe, and various deep Visual disturbances such as contralateral homonymous hemianopsia Diplopia Visual agnosia Cerebral structures including the thalamus and Cortical blindness (if both sides are affected) Artery (PCA) midbrain Superior Upper part of the cerebellum, pons, Cerebellar Contralateral sensory loss Ipsilateral ataxia and cerebral peduncles Artery (SCA) Anterior Inferior Anterior and inferior cerebellum, Vertigo Nausea and vomiting Nystagmus Dysarthria Hearing loss and tinnitus Facial paralysis Cerebellar dorsolateral upper medulla, and and numbness Veering to the ipsilateral side of the lesion and limb ataxia Artery lateral lower pons (AICA) Posterior Inferior Cerebellum Headaches Vertigo Nausea and vomiting Ataxia Cerebellar Artery (PICA) Vertebral and Back of the brain, including the Slurred speech Difficulty swallowing Double vision or vision loss Numbness or tingling Sudden Basilar cerebellum, medulla, midbrain, and falls Vertigo or dizziness Nausea and vomiting Memory loss Loss of consciousness Ataxia Arteries occipital cortex The MCA is the most common site of a stroke due to the direct blood flow from the internal carotid arteries. The PICA is also a classic location for a stroke because it's the first branch off of the vertebral artery. It's important to note that damage to any of these arteries can result in serious complications, including death. Artery Supplies Right and Left Anterior Cerebral Arteries Medial portions of the frontal lobes and superior medial parietal lobes (ACA) Anterior Communicating Artery Connects the two anterior cerebral arteries Right and Left Internal Carotid Arteries Anterior two-thirds of the cerebral hemispheres, including deep white matter and basal ganglia Right and Left Posterior Cerebral Arteries Occipital lobe, the inferior part of the temporal lobe, and various deep structures, including the thalamus and (PCA) the midbrain Right and Left Posterior Communicating Connect the internal carotid arteries to the posterior cerebral arteries Arteries Posterior and medial regions of the cerebral hemispheres, the brainstem, the cerebellum, and cervical spinal Basilar Artery cord Posterior and medial regions of the cerebral hemispheres, the brainstem, the cerebellum, and cervical spinal Vertebral Arteries cord The anterior and posterior communicating arteries connect the anterior and posterior circulations of the brain. These small vessels are important because they provide alternate routes for blood flow if one of the larger arteries is blocked. The internal carotid and vertebral arteries provide the primary blood flow to the brain. The Circle of Willis is a critical structure in the brain that helps to ensure that all parts of the brain receive adequate blood flow. The brain's venous system is responsible for draining oxygen-depleted blood away from the brain and returning it to the body. This system consists of cerebral veins and dural sinuses. Cerebral Veins Cerebral veins drain blood from the brain and empty into the dural sinuses. There are superficial and deep veins. ◦ Superficial veins drain the surfaces of the cerebral hemispheres. ◦ Deep veins drain internal structures. Dural Sinuses Dural sinuses are venous channels located in the dura mater. The dura mater is the tough, outermost layer of the meninges, which are protective membranes surrounding the brain and spinal cord. These sinuses are where blood from the cerebral veins and cerebrospinal fluid (CSF) drain into. Key dural sinuses include: ◦ Superior Sagittal Sinus: The largest dural venous sinus in the brain, it drains blood from the brain's hemispheres. It extends from the frontal bone to the internal occipital protuberance of the occipital bone and empties into the transverse sinuses. ◦ Transverse Sinuses (Lateral Sinuses): Located beneath the brain, these sinuses allow blood to flow from the cerebellum and inferior surfaces of the brain to the back of the brain. They run laterally along the inferior surface of the occipital bone, where they then drain into the sigmoid sinuses. ◦ Sigmoid Sinuses: These are S-shaped, paired dural venous sinuses located beneath the temporal bone. They receive blood from the transverse sinuses and drain into the internal jugular veins, which return blood to the body. Pathway of Venous Drainage The pathway of drainage is from the cerebral veins to the dural sinuses, and then back to the body via the internal jugular veins. Specifically, the pathway is as follows: superior sagittal sinus to the transverse sinuses to the sigmoid sinuses to the internal jugular veins. Clinical Relevance Damage to the sinuses can lead to increased intracranial pressure, potentially resulting in herniation of brain tissue or restricted blood flow. The venous system works in tandem with the arterial system to maintain adequate cerebral blood flow and plays an important role in the overall health and functioning of the brain. The dural sinuses are formed by the separation of the two layers of the dura mater and are located within the dural reflections, including the falx cerebri, tentorium cerebelli, and falx cerebelli. The falx cerebri separates the left and right cerebral hemispheres, while the tentorium cerebelli separates the cerebrum from the cerebellum, and the falx cerebelli separates the left and right hemispheres of the cerebellum. Identify the Cranial Meninges/Understand the Function and Role of Cranial Meninges The cranial meninges are three layers of membranes that cover, protect, and anchor the brain and spinal cord. The meninges also support blood vessels, nerves, and the circulating cerebrospinal fluid (CSF). The three layers of the meninges are the dura mater, the arachnoid mater, and the pia mater. An easy way to remember the order of the meningeal layers is to think of the acronym PAD, P-A-D, which is the order from the inside out: pia mater, arachnoid mater, and dura mater. Dura Mater: ◦ The outermost layer is a thick, tough, and inelastic membrane. It is also referred to as the "tough mother". ◦ The dura mater has two layers: the periosteal layer (closest to the skull) and the meningeal layer (closest to the brain). These layers are mostly fused, but where they separate, they form the dural sinuses. ◦ Dural reflections or folds are formed when the dura mater folds inward on itself. These reflections compartmentalize the brain and limit its movement. The main dural reflections include: ▪ Falx cerebri: A sickle-shaped structure that descends into the longitudinal fissure and separates the left and right cerebral hemispheres. ▪ Tentorium cerebelli: A tent-like structure that separates the cerebrum (temporal and occipital lobes) from the cerebellum and brainstem. It provides a roof over the cerebellum. ▪ Falx cerebelli: A fold of the dura mater that separates the left and right hemispheres of the cerebellum. ◦ The dura mater is attached to the inner surface of the skull and vertebrae. It protects the brain and spinal cord from mechanical trauma and injury. Arachnoid Mater: ◦ The middle layer is a thin, wispy, avascular membrane located beneath the dura mater. It's named for its spider web-like appearance. ◦ The arachnoid mater has trabeculae, connective tissue projections that connect to the pia mater. ◦ It is associated with the cushioning effect with CSF that lies between the arachnoid and pia maters within the subarachnoid space. ◦ The arachnoid mater acts as a barrier to substances entering and leaving the CSF, and it plays a role in the production and reabsorption of CSF. ◦ It bridges over the sulci, which creates CSF-filled triangular spaces. Pia Mater: ◦ The innermost layer is a very delicate, thin membrane that tightly adheres to the surface of the brain and spinal cord. It is also referred to as the "tender mother". ◦ The pia mater is highly vascularized and contributes to the formation of the blood-brain barrier. ◦ It helps to contain CSF and closely follows the contours of all cortical sulci and gyri. Meningeal Spaces: ◦ The meninges create three spaces between their layers: the epidural space, the subdural space, and the subarachnoid space. ◦ Epidural space: Located between the skull and the dura mater. In the spinal cord, this space is between the dura mater and the vertebral bones. The epidural space contains blood vessels, nerve roots, lymphatics, and epidural fat. ◦ Subdural space: Located between the dura mater and the arachnoid mater. It is normally very thin, but can open up due to trauma. ◦ Subarachnoid space: Located between the arachnoid mater and the pia mater. It is filled with CSF, which cushions and protects the brain and spinal cord. This space also houses cranial nerves and intracranial vasculature. In summary, the meninges provide a protective covering for the brain and spinal cord. The dura mater is a tough outer layer, the arachnoid mater is a thin, web-like middle layer, and the pia mater is a delicate inner layer that is in close contact with the brain. These layers and their associated spaces provide both mechanical protection and support for the central nervous system. Identify, describe, and understand the basic pathophysiology of stroke/Appreciate Some of the Diagnostics and Clinical Exams Relevant to Stroke/Introduce Initial Medical Management and Interventions with Acute Stroke There are two main types of stroke: ischemic and hemorrhagic, each with distinct causes and mechanisms. The primary result of either type of stroke is tissue death. Ischemic Stroke Pathophysiology: Ischemic strokes occur when a blood clot or other mass blocks a blood vessel, cutting off the supply of oxygen-rich blood to a particular area of the brain. This blockage leads to a lack of oxygen and nutrients to brain tissue, resulting in an infarct or area of dead tissue. Common Causes: A blood clot that forms elsewhere in the body and travels to the brain, or a clot that develops in an artery that is already narrowed due to atherosclerosis. Reperfusion: The primary treatment goal for ischemic stroke is to restore blood flow to the affected area of the brain as quickly as possible, to try and salvage the penumbra, the area of reversibly injured tissue surrounding the dead core. This can be done through clot-busting medications (tPA or TNK) or surgical removal of the clot (thrombectomy). Hemorrhagic Conversion: A potential complication after an ischemic stroke is hemorrhagic conversion, in which blood vessels in the damaged area rupture after blood flow is restored to the brain, causing bleeding into the brain and potentially worsening outcomes. Hemorrhagic Stroke Pathophysiology: Hemorrhagic strokes happen when a weakened blood vessel, such as an aneurysm, ruptures and spills blood into the brain tissue. The blood puts pressure on the surrounding brain tissue, causing damage. Common Causes: Rupture of an aneurysm, arteriovenous malformations (AVMs), or uncontrolled high blood pressure. Medical Management: Treatment for hemorrhagic stroke focuses on stopping the bleeding and reducing the pressure on the brain. This may include surgery to repair the damaged blood vessel or remove the blood clot. General Information Incidence: Ischemic strokes are more common, accounting for about 87% of all strokes, while hemorrhagic strokes account for approximately 13%. Signs and Symptoms: Common signs and symptoms of stroke, regardless of type, can include sudden weakness or numbness, loss of vision, difficulty speaking or understanding speech, severe headaches with no known cause, dizziness, unsteadiness, or falls. The key feature is the sudden or acute onset of these symptoms. Tissue Death: Both ischemic and hemorrhagic strokes result in tissue death due to either lack of oxygen and nutrients or pressure and damage from blood. It's important to note that both types of strokes can have serious, long-term consequences, including disability, and understanding the differences between the types is important for diagnosis and treatment. Revisit Clinical Presentation and Implications of Stroke with Respect to Cerebral Structures, Functions and Vasculature A stroke is a cerebrovascular event that results in the destruction of brain tissue. It is a leading cause of serious long-term disability. Strokes can be either ischemic or hemorrhagic. Ischemic Stroke Ischemic strokes occur when a clot or mass blocks a blood vessel, cutting off blood flow to a part of the brain. This leads to tissue death. The goal of treating an ischemic stroke is to restore blood flow to the affected area as soon as possible, thereby trying to salvage the penumbra, which is the reversibly injured tissue around the core of dead tissue. Anterior Cerebral Artery (ACA) ◦ The ACA supplies blood to the medial portions of the frontal lobe and the superior medial parietal lobes. ◦ Damage to the ACA can cause contralateral hemiplegia, more so in the leg than the arm. ◦ Other implications of ACA damage include sensory loss, especially in the foot and lower extremity, impaired consciousness, apathy, loss of executive functions, frontal lobe behavior changes, speech dysfunctions, and urinary incontinence. Middle Cerebral Artery (MCA) ◦ The MCA supplies a large portion of the lateral cerebral cortex, including the frontal, temporal, and parietal lobes. ◦ It is vital for motor and sensory functions of the face, throat, hand, and arm, as well as speech and language processing. ◦ Damage to the MCA can result in contralateral hemiplegia, more so in the arm and face than the leg. ◦ Other implications of MCA damage include sensory loss (including vision, hearing, smell, taste, and touch), aphasia (if in the dominant hemisphere) or dysarthria, swallowing issues, hemineglect or inattention (if in the non-dominant hemisphere), and vision deficits. ◦ The MCA is the blood vessel most affected by strokes, due to its direct flow from the internal carotid artery. Posterior Cerebral Artery (PCA) ◦ The PCA supplies the occipital lobe, the inferior part of the temporal lobe, and various deep structures, including the thalamus and midbrain. ◦ It is essential for visual processing. ◦ Damage to the PCA can result in visual disturbances, such as contralateral homonymous hemianopsia, diplopia, visual agnosia, or cortical blindness. Cerebellar and Brainstem Vasculature ◦ The vertebral and basilar arteries supply blood to the back of the brain, which controls functions such as breathing, heart rate, vision, movement, and balance. ◦ Occlusion of or damage to these arteries can result in slurred speech, difficulty swallowing, double vision, numbness or tingling, sudden falls, vertigo or dizziness, nausea and vomiting, memory loss, loss of consciousness, and ataxia. ◦ Superior Cerebellar Artery: Damage can cause contralateral sensory loss and ipsilateral ataxia. ◦ Anterior Inferior Cerebellar Artery: Damage can cause vertigo, nausea, vomiting, nystagmus, dysarthria, hearing loss, tinnitus, facial paralysis and numbness, and ataxia. ◦ Posterior Inferior Cerebellar Artery (PICA): Damage can cause headache, vertigo, nausea, vomiting and ataxia. The PICA is the most common location for a stroke in the posterior circulation. Hemorrhagic Stroke Hemorrhagic strokes occur when a weakened blood vessel ruptures, spilling blood into the brain. This leads to tissue damage due to the pressure from the blood on surrounding brain tissue. Medical management for a hemorrhagic stroke focuses on repairing or removing the source of bleeding in the brain. General Signs and Symptoms of Stroke Sudden weakness or numbness in the face, arm, or leg Sudden loss of vision, particularly in one eye Sudden difficulty speaking or understanding speech Sudden severe headaches with no known cause Sudden unexplained dizziness, unsteadiness, or falls The sudden or acute onset of these symptoms is a key indicator of a vascular problem Clinical Implications Understanding the relationship between the affected blood vessels and the specific brain regions is essential for diagnosing and treating strokes. For instance, a stroke in the MCA territory will likely affect motor and sensory functions of the face and arm, while a stroke in the PCA territory may primarily affect vision. The NIH Stroke Scale is a standard neurological exam completed on stroke patients within the first 48 hours, and higher scores correlate with more severe strokes. The NIH Stroke Scale is used to assess the severity of the stroke and predict clinical outcomes, but does not serve as the primary guide for tPA administration. Imaging, such as a head CT without contrast, is used to differentiate between hemorrhagic and ischemic strokes, and to guide medical interventions. A CT scan is the fastest and most convenient test for stroke diagnosis. MRI has greater resolution and can detect cerebral ischemia earlier, but is not always immediately available. tPA (tissue plasminogen activator) is a clot-busting medication used to treat ischemic strokes and must be administered within a specific time window of symptom onset. A thrombectomy, which is the surgical removal of a blood clot, may be appropriate for patients with an ischemic stroke. Hemorrhagic conversion is a complication that can occur after an ischemic stroke, in which blood vessels rupture, leading to bleeding in the brain. In summary, the clinical presentation of a stroke is directly related to the affected cerebral structures, their functions, and the specific vasculature involved. Recognizing these relationships is critical for effective diagnosis, treatment, and rehabilitation of stroke patients. Introduce the Anatomy of Ventricles in the Brain The brain's ventricles are a network of cavities within the brain that are filled with cerebrospinal fluid (CSF). There are four ventricles: two lateral ventricles, and the third and fourth ventricles. The ventricles produce and secrete CSF, as well as transport and remove it from the cranial cavity, protecting and supporting the brain. Lateral Ventricles: The two largest ventricles, located in the cerebrum, are C-shaped with a central body in the parietal lobe. They also have three horns that extend into different lobes of the brain: the anterior horn (frontal lobe), the inferior horn (temporal lobe), and the posterior horn (occipital lobe). The two lateral ventricles are separated by the septum pellucidum. The lateral ventricles connect to the third ventricle via the interventricular foramen of Monro. Third Ventricle: A narrow, slit-like, vertical cavity located in the diencephalon between the right and left thalami. The third ventricle connects to the lateral ventricles via the interventricular foramen of Monro and to the fourth ventricle via the cerebral aqueduct. Cerebral Aqueduct: This is a connecting structure that carries CSF from the third ventricle to the fourth ventricle, running through the midbrain. It is also known as the Aqueduct of Sylvius and is the thinnest part of the ventricular system. Fourth Ventricle: A large, tent-like chamber located in the hindbrain (brainstem) at the back of the pons and the upper half of the medulla oblongata. It is continuous with the cerebral aqueduct and the central canal of the spinal cord. The fourth ventricle connects to the subarachnoid space and has three openings for CSF circulation: two lateral apertures (foramen of Luschka) and one median aperture (foramen of Magendie). CSF flows in a unidirectional path, starting in the lateral ventricles, then moving through the foramen of Monro to the third ventricle, then through the cerebral aqueduct to the fourth ventricle. From the fourth ventricle, the CSF exits into the subarachnoid space of the brain and spinal cord via the foramens of Luschka and Magendie. Finally, the CSF is reabsorbed into the bloodstream in the sagittal sinus. CSF is constantly produced, circulated, and reabsorbed, helping to cushion and protect the central nervous system while clearing out toxins and waste. The choroid plexus, located in each ventricle and the central canal, is a network of capillaries and specialized epithelial tissue called ependyma that produces CSF. The choroid plexus also forms the blood-CSF barrier, which is similar to the blood-brain barrier. Discuss Ventricular Function and Purpose The ventricles of the brain are a communicating network of cavities filled with cerebrospinal fluid (CSF). The primary functions of the ventricles are to produce and secrete CSF, to transport and remove CSF around and from the cranial cavity, and to protect and support the brain. Here is more detail about the function and purpose of the ventricles: CSF Production: The choroid plexus, a network of capillaries and specialized epithelial tissue called ependyma located within the pia mater, is found in each of the four ventricles and the central canal and is primarily responsible for producing CSF. The ciliated ependyma line the ventricles and central canal. CSF Circulation: The ventricles facilitate the circulation of CSF through the central nervous system. The CSF flows in a specific, unidirectional path: from the lateral ventricles to the third ventricle through the interventricular foramen of Monro, then through the cerebral aqueduct to the fourth ventricle. From there, the CSF exits into the subarachnoid space of the brain and spinal cord via the foramens of Luschka and foramen of Magendie. Finally, the CSF is reabsorbed into the bloodstream in the sagittal sinus. This constant circulation ensures that the brain and spinal cord are bathed in CSF. Protection and Support: CSF acts as a cushion, protecting the brain and spinal cord from mechanical trauma and injury. CSF also helps to clear out toxins and waste products released by nerve cells and delivers nutrients to the central nervous system. Blood-CSF Barrier: The choroid plexus, along with the arachnoid mater, forms the blood-CSF barrier, which separates blood from CSF. This barrier is similar to the blood-brain barrier and prevents harmful bloodborne substances from entering the brain while facilitating the exchange and removal of metabolites. Identify Related Clinical Conditions and Ventricular Pathology Clinical conditions related to ventricular pathology primarily involve disruptions in the normal flow or production of cerebrospinal fluid (CSF), leading to conditions such as hydrocephalus. Hydrocephalus: This condition occurs when CSF builds up in the brain, causing the brain's ventricles to widen and put pressure on the brain tissue. It is also known as "water on the brain". ◦ Causes: Hydrocephalus can result from a variety of factors: ▪ Infection: Meningitis and ventriculitis can lead to inflammation and block CSF flow. ▪ Head Trauma: Injuries to the head can disrupt normal CSF circulation. ▪ Brain Tumors: Tumors can obstruct the ventricular system, causing CSF to accumulate. ▪ Brain Bleeds: Hemorrhages can cause blockages and impact CSF flow. ▪ Genetic Problems: Gene mutations can lead to hydrocephalus. ▪ Birth Defects: Conditions like Spina bifida and Chiari malformation can cause hydrocephalus. ◦ Normal Pressure Hydrocephalus (NPH): A rare neurological condition that results from CSF buildup in the brain. Symptoms of NPH can develop over time and are similar to those of Parkinson's or Alzheimer's disease. ◦ Pathophysiology: Inflammation or blockages in the brain's anatomy can impede the normal flow of CSF, resulting in swollen ventricles. This buildup of CSF leads to increased pressure on the brain tissue. ◦ Symptoms: Symptoms of hydrocephalus can include: ▪ Headaches ▪ Nausea and vomiting ▪ Lethargy or sleepiness ▪ Dizziness ▪ Blurred vision ▪ Loss of bladder control ▪ Confusion or forgetfulness ▪ Loss of balance, and problems with coordination or walking ▪ Speech deficits ▪ Mood changes or depression ◦ Diagnosis: Hydrocephalus is typically diagnosed using imaging techniques such as MRI or head CT scans, which allow visualization of the ventricles to identify any abnormalities. ◦ Treatment: Interventions for hydrocephalus include: ▪ Surgery to place a shunt to drain excess CSF ▪ Medications or other procedures to remove the excess CSF ▪ Ventriculoperitoneal (VP) shunt: This shunt diverts CSF from the brain to the abdomen. ▪ Extraventricular drain: This temporary fix drains CSF from the brain to a system outside the body. Other Considerations: Persistent hydrocephalus can lead to permanent brain damage and can be fatal if left untreated. Week 3 Describe the 3 Main Structures within the Brainstem and Their Function Brainstem Functions Structure The midbrain is the most superior or rostral component of the brainstem. It is involved in cranial nerve function, specifically cranial nerves 3 and 4, which are responsible for eye movement. The midbrain contains the red nucleus, which helps facilitate motor coordination and control, especially Midbrain with walking. The substantia nigra, also in the midbrain, produces dopamine, which influences movement. Damage to the midbrain can result in decorticate or decerebrate posturing. The pons is inferior to the midbrain and superior to the medulla. It houses the nuclei of cranial nerves V, VI, VII, and VIII. The pons is Pons embryologically linked to the cerebellum. The medulla oblongata is the most inferior or caudal structure of the brainstem. It is superior to the spinal cord. The medulla houses the nuclei of Medulla cranial nerves IX, X, XI, and XII. The medulla also contains the olivary nuclei, which aid in cerebellar function and perception of sound. It is the location where many vertical tracts decussate or cross, which is a key feature for movement and sensation. Define the Reticular Activating System and Its Influence on Human Movement The reticular activating system (RAS) is a component of the reticular formation, which is a loose aggregate of cells that spans the brainstem and extends up to the thalamus. The reticular formation and, specifically, the RAS, plays a role in several functions, including integrating sensory information, regulating motor and autonomic functions, and modulating pain. The RAS is crucial for generating conscious levels of awareness. Here are some key points about the reticular activating system's influence on human movement: Regulation of Neuronal Activity: The RAS contains various nuclei that regulate neuronal activity throughout the central nervous system by producing specific neurotransmitters. For example, the raphe nuclei produces serotonin, and the locus coeruleus and medial reticular area produce norepinephrine, both of which influence other parts of the brainstem, cerebrum and cerebellum. These neurotransmitters have a significant influence on motor activity. General Awareness: The RAS is involved in a general awareness of self and surroundings. The reticular formation integrates different sensory information from throughout the nervous system. This general awareness can impact an individual's movement. Motor Regulation: The reticular formation is involved in regulating motor functions. Although it is not fully vetted, there is evidence to suggest that the reticular formation is involved in locomotion. The reticular formation helps to prime the body for movement. Levels of Consciousness: The RAS is involved in governing alertness, sleep, and attention. Because of this, damage to the RAS can result in different levels of consciousness, which in turn will impact an individual's ability to move. For example, injuries to the brainstem can lead to disorders of consciousness, ranging from a coma to a minimally conscious state. The RAS works by maintaining various bodily functions and influencing higher-level brain processes. During a movement, such as sprinting, the reticular formation "lights up" in order to prime the body. Recall Relevant Blood Supply to the Brainstem The brainstem's blood supply is crucial for its function, and several major arteries contribute to this supply. The specific arteries that provide blood to the different structures of the brainstem include: Medulla: The medulla receives blood from the vertebral branches, which include the anterior spinal artery and the posterior spinal artery. Pons: The pons is supplied by the basilar artery and its branches, particularly the pontine arteries. Midbrain: The midbrain receives blood from the posterior cerebral artery and the posterior communicating artery. It's important to note that many brainstem issues are vascular in nature, meaning that they are caused by problems with blood supply. Because of this, an insult to the brainstem will often present fairly quickly. This rapid onset is a key indication that the issue is likely vascular, rather than a slower onset issue like a tumor or degenerative disease. The brainstem's critical role means that injuries to its blood supply can have severe consequences, leading to varying levels of consciousness or disorders of consciousness. These disorders can range from a coma to a minimally conscious state. Define the 12 Cranial Nerves and Their Function/Explain the Consequences of Lesion to Each Sensory, Primary Ipsilateral or Cranial Nerve Start Location End Location Function How to Test Motor, or Nuclei Contralateral Mixed Nasal cavity at Olfactory cortex Have the patient Olfactory I. Olfactory the cribriform along the temporal Smell identify familiar Sensory Clinically insignificant bulb plate bone scents like coffee Lateral Ipsilateral before the Primary visual geniculate Eye chart, Posterior orbit optic chiasm, II. Optic cortex of the nucleus of Visual acuity peripheral vision Sensory of the eye contralateral in the occipital lobe the tests posterior aspect thalamus Primarily Medial rectus, Oculomotor Eye movements up, down, and Eye movement Motor with superior rectus, nucleus and medially; torsion; elevation of assessment, autonomic III. Oculomotor Midbrain levator palpebrae Edinger- Ipsilateral upper eyelid; pupillary pupillary light function for superioris, inferior Westphal constriction reflex pupillary rectus nucleus constriction Contralateral from a Convergence test, Inferior lesion in the Superior oblique Trochlear Depresses the eye, especially observation of IV. Trochlear colliculus of the Motor brainstem, ipsilateral muscle nucleus when adducted resting eye midbrain from a lesion on the position nerve itself Sensory: Light touch on