Developmental Biology Unit 1 PDF
Document Details
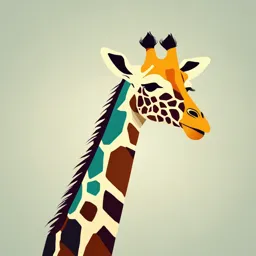
Uploaded by PermissibleMossAgate2914
SRM Institute of Science and Technology
Sylvia S. Mader
Tags
Summary
This document provides an overview of key concepts in developmental biology, including the stages of development of multicellular organisms, the importance of model organisms, and the relationships between life cycles and evolution in biology.
Full Transcript
Unit-1 Levels of Organizatio n Inquiry into Life, Sylvia S. Mader, McGraw-Hill Companies, 11th edition Stages of Development of an organism Development is the process by which a multicellular organism, beginning with a single cell, goes through a series of changes, taking on...
Unit-1 Levels of Organizatio n Inquiry into Life, Sylvia S. Mader, McGraw-Hill Companies, 11th edition Stages of Development of an organism Development is the process by which a multicellular organism, beginning with a single cell, goes through a series of changes, taking on the successive forms that characterize its life cycle https://digfir-published.macmillanusa.com/pol2e/pol2e_ch14_2.html The fertilized egg is known as zygote, and the organism developing in the time between the fertilization and birth is known as embryo The study of development of an embryo is defined as embryology The study of all the processes that occur in an organism from embryo to adulthood, including regeneration, asexual reproduction, metamorphosis, and stem cell growth is known as Developmental Biology. Two fundamental questions in developmental biology How does the zygote give rise to the adult body? how does the adult body produce yet another body? Developmental Biology 13e By Michael Barresi, Scott Gilbert Considerations to choose a model organism In order to answer the question related to development, researchers should always think what makes an organism as a “good model” for addressing a given question? Different organisms provide the researcher with different advantages, however there are certain considerations to be considered, some of them includes: Size: It must be feasible to house a significant number of breeding adults in the laboratory.. Generation time: How long does it take the organism to complete its life cycle from embryo to reproductive adult? Additionally, how short is the embryonic period? Embryo accessibility: To study embryology, a researcher needs to be able to see and work with actual embryos, preferably many of them. Different species pose different challenges for embryo accessibility. Organism type and phylogenetic position: Ideally, the research question should guide the selection of a model system. A researcher interested in metamorphosis is limited to species that display these remarkable transformations, such as frogs. Life cycles and the evolution of developmental patterns The view taken here is that the life cycle is the central unit in biology. … Evolution then becomes the alteration of life cycles through time, genetics the inheritance mechanisms between cycles, and development all the changes in structure that take place during one life cycle. Gilbert, Developmental Biology An animal’s life cycle The stages of development between fertilization and hatching or birth are collectively called embryogenesis. Most animals pass through similar stages of development: fertilization, cleavage, gastrulation, organogenesis, hatching or birth, metamorphosis, and gametogenesis. Fertilization gametogenesis Cleavag e Gastrulation Metamorphosis Hatching or birth Organogenesis An animal’s life cycle Most animals pass through similar stages of development: fertilization, cleavage, gastrulation, organogenesis, hatching or birth, metamorphosis, and gametogenesis. The stages of development between fertilization and hatching or birth are collectively called embryogenesis. 1. Fertilization involves the fusion of the mature sex cells, the sperm and egg, which are collectively called the gametes. Fusion of the gametes stimulates the egg to begin development, initiating a new individual. The subsequent fusion of the gamete nuclei gives the embryo its genome, that helps the embryo to develop in a manner very similar to that of its parents. 2. Cleavage is a series of mitotic divisions that immediately follow fertilization. During cleavage, the enormous volume of zygote cytoplasm is divided into numerous smaller cells called blastomeres. By the end of cleavage, the blastomeres have usually formed a sphere, known as a blastula. 3. After the rate of mitotic division slows down, the blastomeres undergo dramatic movements and change their positions relative to one another. This series of extensive cell rearrangements is called gastrulation, and the embryo is said to be in the gastrula stage. 4. Organogenesis: As a result of gastrulation, the embryo contains three germ layers (the endoderm, ectoderm, and mesoderm). Once the germ layers are established, the cells interact with one another and rearrange themselves to produce the tissues and organs of the body. Chemical signals are exchanged between cells of the germ layers, resulting in the formation of specific organs at specific sites. This process is called organogenesis. Certain cells undergo long migrations from their place of origin to their final location; such migrating cells include the precursors of blood cells, lymph cells, pigment cells, and gametes (eggs and sperm). 5. In addition to establishing which cells will be in which germ layer, early embryos develop three crucial axes that are the foundation of the body. The anterior-posterior axis extends from head to tail. The dorsal-ventral axis extend from back (Latin, dorsum) to belly (ventrum). The right-left axis separates the lateral sides of the body. Although humans (for example) may look symmetrical, in most of us, the heart is in the left half of the body, while the liver is on the right. Somehow, the embryo “knows” that some organs belong on one side or the other. 6. In most species, the organism that hatches from the egg or is born into the world is not sexually mature, but undergoes growth, maturation, and sometimes complete metamorphosis to become a sexually mature adult. 7. In many species, a group of cells in the developing embryo is set aside to produce the next generation of embryos. These cells are the precursors of the gametes. The gametes and their precursor cells are collectively called germ cells, and they are set aside for reproductive function. 8. The germ cells eventually migrate to the gonads, where they differentiate into gametes. The development of gametes, called gametogenesis, is usually not completed until the organism has become physically mature. At maturity, the gametes may be released and participate in fertilization to begin a new embryo. The adult organism eventually undergoes senescence and dies, its nutrients often supporting the early embryogenesis of its offspring and its absence allowing less competition. Thus, the cycle of life is renewed. A frog’s life cycle Life cycles are often controlled by environmental factors. E.g., Frog (Rana) In most of the frogs, gametogenesis and fertilization are seasonal events. A combination of photoperiod and temperature informs the pituitary gland of mature female frog that the season is spring and pituitary hormones cause gametes to mature. Usually, Rana pipiens lay their eggs in pond vegetation and the egg jelly adhers to the plants and anchors the eggs (another example of importance of environment factors in life cycle). Fertilization In frogs, fertilization is external. Male frog clasps the female’s back and fertilize the eggs as the female releases them. Rana pipiens lays about 6500 eggs in early spring. Fertilization accomplishes both sex (genetic recombination) and reproduction (the generation of new individuals). The genomes of the haploid male and female pronuclei merge and recombine to form the diploid zygote nucleus. The entry of the sperm facilitates the movement of cytoplasm inside the newly fertilized egg. This cytoplasmic migration is critical in determining the three body axes. Fertilization activates the molecules necessary to initiate cell cleavage and gastrulation newly laid clutch of eggs 8-cell embryo Cleavage and Gastrulation During cleavage, the volume of the frog zygote stays the same, but it divides into tens of thousands of cells. Cleavage is regulated by proteins and mRNAs that have been stored in the oocyte cytoplasm. Later in cleavage, the zygote genome becomes activated and produces the products needed for further development, including those needed for gastrulation. Gastrulation in frogs begins at a point on the embryo surface roughly 180° opposite the point of sperm entry, with the formation of a dimple called the blastopore. 8-cell embryo A late blastula, containing An early gastrula thousands of cells The initial site of the blastopore, which marks the future dorsal (spinal) side of the embryo, expands to become a ring. Cells migrating through the blastopore enter the embryo’s interior, while cells remaining on the embryo’s exterior become the ectoderm, which expands to enclose the entire embryo. Thus, at the end of gastrulation, the ectoderm covers the outside of the embryo, the gut-producing endoderm is deep inside the embryo, and the organ-forming mesoderm is between the endoderm and ectoderm. Organogenesis Vertebrate organogenesis begins when the cells of the most dorsal region of the mesoderm condense to form the rod of cells called the notochord. These notochord cells produce chemical signals that redirect the fate of the ectodermal cells above it. Instead of forming epidermis, the cells above the notochord are instructed to become the cells of the nervous system. The cells change shape and rise up from the round body. At this stage, the embryo is called a neurula. The neural precursor cells elongate, stretch, and fold into the embryo, forming the neural tube. The future epidermal cells of the back cover the neural tube. A neurula, where the neural folds come together at the dorsal midline, creating a neural tube The mesodermal tissue adjacent to the neural tube and notochord becomes segmented into somites—the precursors of the frog’s back muscles, spinal vertebrae, and dermis (the inner portion of the skin). The embryo develops a mouth and an anus, and it elongates into the familiar tadpole structure. The neurons make connections to the muscles and to other neurons, the gills form, and the larva is ready to hatch from its egg. The hatched tadpole will feed for itself as soon as the yolk supplied by its mother is exhausted. METAMORPHOSIS Metamorphosis of a fully aquatic tadpole larva into an adult frog that can live on land is one of the most striking transformations in all biology. The hindlimbs and forelimbs of adult will use for locomotion differentiate as the tadpole’s paddle tail recedes. The cartilaginous tadpole skull is replaced by the predominantly bony skull of the young frog The horny teeth - the tadpole uses to tear up pond plants disappear, the mouth and jaw take a new shape, and the fly-catching tongue muscle of the frog develops. The tadpole’s lengthy intestine—characteristic of herbivores—shortens to suit the more carnivorous diet of the adult frog. The gills regress and the lungs enlarge. Amphibian metamorphosis is initiated by hormones from the tadpole’s thyroid gland. An adult leopard frog can burrow into the mud and survive the winter; its tadpole cannot. GAMETOGENESIS As metamorphosis ends, the development of the germ cells (sperm and eggs) begins. To become mature, the germ cells must be competent to complete meiosis, the cell divisions that halve the number of chromosomes to produce haploid gametes. Having undergone meiosis, the mature sperm and egg nuclei can unite in fertilization, restoring the diploid chromosome number and initiating the events that lead to development and the continuation of the circle of life. Premetamorphic tadpole Prometamorphic tadpole, showing hindlimb growth Onset of metamorphic climax as forelimbs emerge. adult frog A flowering plant’s life cycle Arabidopsis (consider the mustard seed) It completes its life cycle in only 6 weeks, techniques for its propagation are routine, and its comparatively small genome has been fully sequenced and annotated. REPRODUCTIVE AND GAMETOPHYTIC PHASES An adult flowering plant in the reproductive phase has fully developed sporocyte flowers with pollen-producing stamens (male reproductive organs) and ovary-containing carpels (female reproductive organs). These produce the haploid sperm and egg, respectively. These gametes and their associated haploid cells are produced in the gametophytic phase. When pollen carrying the sperm delivers sperm to eggs in the ovary, fertilization occurs, yielding a diploid zygote (single-celled embryo). EMBRYOGENESIS AND SEED MATURATION In seed-producing plants, cleavage is not constrained by yolk, as the embryo’s nutrient supply comes from the surrounding endosperm in the seed. The zygote divides asymmetrically. The first cell division yields large basal cell and a much smaller apical cell. The apical cell will generate the embryo proper, while the basal cell becomes the suspensor that supports the embryo. This initial asymmetrical division sets up the primary (apical-basal) axis of the embryo, such that shoots (stem, leaves, and flowers) will grow from the apicalmost cells, while roots will develop from the basalmost cells (i.e., the embryonic cells closest to the suspensor). MERISTEMS AND TISSUE TYPES Although plants do not have the huge variety of cell and tissue types seen in many animals, three distinct tissue types immediately become segregated in the embryo: dermal tissue, ground tissue, and vascular tissue. The dermal cells produce the outer layers of the plant epidermis. Ground tissue cells make up the bulk of a plant’s internal structures. The cells at the very core of the embryo form the vascular tissues: xylem, the vessels that carry water and nutrients upward through the plant; and phloem, which carries the sugars produced by photosynthesis, along with other metabolites. Phloem transport is primarily from the leaves to those parts of the plant that consume more energy and nutrients than they produce. VEGETATIVE PHASES: FROM SPOROPHYTIC GROWTH TO INFLORESCENCE IDENTITY Upon completion of germination, the now-established sporophyte grows. This marks the beginning of the juvenile vegetative phase, which generally increases the plant’s mass and overall size as it continues into the adult vegetative phase. The next phase is the adult reproductive phase, during which a change in the differentiation program of the shoot meristem cells occurs. Instead of generating leaves and stems, the plant starts producing flowers, with their gametophytic lineages of reproductive tissues. The life cycle is poised to repeat. Principles of Development: Life Cycles and Developmental Patterns 1. The life cycle can be considered a central unit in biology. The adult form need not be paramount. In a sense, the life cycle is the organism. 2. The basic life cycle consists of fertilization, cleavage, gastrulation, germ layer formation, organogenesis, metamorphosis, adulthood, and senescence 3. Reproduction need not be sexual. Some organisms, such as Volvox and Dictyostelium, exhibit both asexual reproduction and sexual reproduction. 4. Cleavage divides the zygote into numerous cells called blastomeres. 5. In animal development, gastrulation rearranges the blastomeres and forms the three germ layers. 6. Organogenesis often involves interactions between germ layers to produce distinct organs. 7. Germ cells are the precursors of the gametes. Gametogenesis forms the sperm and the eggs. 8. There are three main ways to provide nutrition to the developing embryo: (1) supply the embryo with yolk; (2) form a larval feeding stage between the embryo and the adult; or (3) create a placenta between the mother and the embryo. Principles of Development: Life Cycles and Developmental Patterns 9. Life cycles must be adapted to the nonliving environment and interwoven with other life cycles. 10. Don't regress your tail until you've formed your hindlimbs. 11. Protostomes and deuterostomes represent two different sets of variations on development. Protostomes form the mouth first, while deuterostomes form the anus first. Principles of experimental Embryology Courtesy: Scott F. Gilbert, Michael J. F. Barresi. Developmental Biology, Sinauer Associates Oxford University Press Principles of experimental embryology Three major research programs in experimental embryology In this topic, we will discuss three of the major research programs in experimental embryology. The first concerns how forces outside the embryo influence its development. The second concerns how forces within the embryo cause the differentiation of its cells. The third looks at how the cells order themselves into tissues and organs. 1. How forces outside the embryo influence its development? (Environmental sex determination) Environment plays a major role in the development of an organism Early embryologists: Alter environment to see if affect phenotype of embryo Environment could play a role in the ways genes respond and organism develops How forces outside the embryo influence its development? (Environmental sex determination) SEX DETERMINATION IN A VERTEBRATE: ALLIGATOR. Recent research has shown that the sex of alligators, crocodiles, and many other reptiles depends not on chromosomes, but on temperature. After studying the sex determination of the Mississippi alligator both in the laboratory and in the field, Ferguson and Joanen (1982) concluded that sex is determined by the temperature of the egg during the second and third weeks of incubation. Eggs incubated at 30°C or below during this time period produce female alligators, whereas those eggs incubated at 34°C or above produce males (At 32°C, 87% of the hatchlings were female). On the other hand, nests built in wet marshes (close to 30°C) produce females, nests constructed on levees (close to 34°C) give rise to males. SEX DETERMINATION IN AN ECHIUROID WORM: BONELLIA. When the field of developmental mechanics was first formulated, some of the obvious variables to manipulate were the temperature and media in which embryos were developing. Baltzer (1914) showed that the sex of the echiuroid worm Bonellia viridis depended on where the larva settled. The female Bonellia worm is a marine, rock-dwelling animal, with a body about 10 cm long. She has a proboscis that can extend over a meter in length. The male Bonellia, however, is only 1–3 mm long and resides within the uterus of the female, fertilizing her eggs. Baltzer showed that if a Bonellia larva settles on the seafloor, it becomes a female. However, should a larva land on a female’s proboscis (which apparently emits chemical signals that attract larvae), it enters the female’s mouth, migrates into her uterus, and differentiates into a male. Thus, if a larva lands on the seafloor, it becomes female; if it settles on a proboscis, it becomes male. Adaptation of embryos and larvae to their environments (Phenotypic plasticity) Another program of environmental developmental biology concerns how the embryo adapts to its particular environment. August Weismann (1875) pioneered the study of larval adaptations, and recent research in this area has provided some fascinating insights into how an organism’s development is keyed to its environment. Weismann noted that butterflies that hatched during different seasons were colored differently, and that this season-dependent coloration could be mimicked by incubating larvae at different temperatures. Phenotypic variants that result from environmental differences are often called morphs. One example of such seasonal variation is the European map butterfly, Araschnia levana, which has two seasonal phenotypes so different that Linnaeus classified them as two different species. The spring morph is bright orange with black spots, while the summer morph is mostly black with a white band. summer morph spring morph 2. How do forces INSIDE the embryo encourage cells to differentiate? The development of specialized cell types is called differentiation. These overt changes in cellular biochemistry and function are preceded by a process resulting in the cell commitment to a certain fate. The process of commitment can be divided into two stages Specification - The fate of a cell or a tissue is said to be specified when it is capable of differentiating autonomously when placed in a neutral environment, such as a petri dish or test tube. At this stage, the commitment is still reversible. Determination - A cell or tissue is said to be determined when it is capable of differentiating autonomously even when placed into another region of the embryo. Here, the cell commitment is irreversible Autonomous specification In this case, if a particular blastomere is removed from an embryo early in its development, that isolated blastomere will produce the same types of cells that it would have made if it were still part of the embryo Autonomous specification of trochoblast (ciliated) cells of the snail Patella. (A) 16-Cell stage seen from the side; presumptive trochoblast cells are pink. (B) 48-Cell stage. (C) Ciliated larval stage, seen from the animal pole. (D–G) Differentiation of a Patella trochoblast cell isolated from the 16-cell stage and cultured in vitro. Even in isolated culture, the cells divide and become ciliated at the correct times, indicative of autonomous specification. (After E. B. Wilson. 1904. J Exp Zool 1: 1–72.) Autonomous specification gives rise to a pattern of embryogenesis referred to as mosaic development, since the embryo appears to be constructed like a tile mosaic of independent, self-differentiating parts. Invertebrate embryos, especially those of molluscs, annelids, and tunicates, often use autonomous specification to determine the fates of their cells. In these embryos, morphogenetic determinants (certain proteins or messenger RNAs) are placed in different regions of the egg cytoplasm and are apportioned to the different cells as the embryo divides. These morphogenetic determinants specify the cell type. Autonomous specification was first demonstrated in 1887 by a French medical student, Laurent Chabry. Conditional specification A second mode of commitment involves interactions among neighbouring cells is conditional specification. In this type of specification, each cell originally has the ability to become any of many different cell types. However, interactions of the cell with other cells restrict the fate of one or more of the participants. This mode of commitment is sometimes called conditional specification because the fate of a cell depends upon the conditions in which the cell finds itself. If a blastomere is removed from an early embryo that uses conditional specification, the remaining embryonic cells alter their fates so that the roles of the missing cells can be taken over. This ability of embryonic cells to change their fates to compensate for the missing parts is called regulation. The isolated blastomere can also give rise to a wide variety of cell types (and sometimes generates cell types that the cell would normally not have made if it were still part of the embryo). Thus, conditional specification gives rise to a pattern of embryogenesis called regulative development. Regulative development is seen in most vertebrate embryos, and it is obviously critical in the development of identical twins. Syncytial specification A third mode of specification uses elements of both autonomous and conditional specification. A cytoplasm that contains many nuclei is called a syncytium, and the specification of presumptive cells within a syncytium is syncytial specification. Insects are notable examples of embryos that go through a syncytial stage, as illustrated by the fruit fly Drosophila melanogaster. During the fly’s early cleavage stages, nuclei divide through 13 cycles in the absence of any cytoplasmic cleavage. This division creates an embryo of many nuclei contained within one shared cytoplasm surrounded by one common cell membrane, called a syncytial blastoderm 3. How the cells order themselves into tissues and organs? A body is more than a collection of randomly distributed cell types. Development involves not only the differentiation of cells, but also their organization into multicellular arrangements such as tissues and organs. How can matter organize itself so as to create a complex structure such as a limb or an eye? There are five major questions for embryologists who study morphogenesis: 1. How are tissues formed from populations of cells? For example, how do neural retina cells stick to other neural retina cells rather than becoming integrated into the pigmented retina or iris cells next to them? How are the various cell types within the retina (the three distinct layers of photoreceptors, bipolar neurons, and ganglion cells) arranged so that the retina is functional? 2. How are organs constructed from tissues? The retina of the eye forms at a precise distance behind the cornea and the lens. The retina would be useless if it developed behind a bone or in the middle of the kidney. Moreover, neurons from the retina must enter the brain to innervate the regions of the brain cortex that analyze visual information. All these connections must be precisely ordered. 3. How do organs form in particular locations, and how do migrating cells reach their destinations? Eyes develop only in the head and nowhere else. What stops an eye from forming in some other area of the body? Some cells—for instance, the precursors of our pigment cells, germ cells, and blood cells—must travel long distances to reach their final destinations. How are cells instructed to travel along certain routes in our embryonic bodies, and how are they told to stop once they have reached their appropriate destinations? 4. How do organs and their cells grow, and how is their growth coordinated throughout development? The cells of all the tissues in the eye must grow in a coordinated fashion if one is to see. Some cells, including most neurons, do not divide after birth. In contrast, the intestine is constantly shedding cells, and new intestinal cells are regenerated each day. The mitotic rate of each tissue must be carefully regulated. If the intestine generated more cells than it sloughed off, it could produce tumorous outgrowths. If it produced fewer cells than it sloughed off, it would soon become nonfunctional. What controls the rate of mitosis in the intestine? 5. How do organs achieve polarity? If one were to look at a cross section of the fingers, one would see a certain organized collection of tissues—bone, cartilage, muscle, fat, dermis, epidermis, blood, and neurons. Looking at a cross section of the forearm, one would find the same collection of tissues. But they are arranged very differently in different parts of the arm. How is it that the same cell types can be arranged in different ways in different parts of the same structure? Differential cell affinity Each type of cell has a different set of proteins at its surfaces, and that some of these differences are responsible for forming the structure of the tissues and organs during development. Observations of fertilization and early embryonic development made by E. E. Just (1939) suggested that the cell membrane differed among cell types. The modern analysis of morphogenesis began with the experiments of Townes and Holtfreter in 1955. Taking advantage of the discovery that amphibian tissues become dissociated into single cells when placed in alkaline solutions, they prepared single-cell suspensions from each of the three germ layers of amphibian embryos soon after the neural tube had formed. Two or more of these single-cell suspensions could be combined in various ways. When the pH of the solution was normalized, the cells adhered to one another, forming aggregates on agar-coated petri dishes. By using embryos from species having cells of different sizes and colors, Townes and Holtfreter were able to follow the behavior of the recombined cells. The results of their experiments were striking. First, they found that reaggregated cells become spatially segregated. That is, instead of the two cell types remaining mixed, each cell type sorts out into its own region. Thus, when epidermal (ectodermal) and mesodermal cells are brought together to form a mixed aggregate, the epidermal cells move to the periphery of the aggregate and the mesodermal cells move to the inside. In no case do the recombined cells remain randomly mixed, and in most cases, one tissue type completely envelops the other. Second, the researchers found that the final positions of the reaggregated cells reflect their embryonic positions. Sorting out and reconstruction of spatial relationships in aggregates of embryonic amphibian cells. (After Townes and Holtfreter 1955.) The third conclusion of Holtfreter and his colleagues was that selective affinities change during development. Such changes should be expected, because embryonic cells do not retain a single stable relationship with other cell types. For development to occur, cells must interact differently with other cell populations at specific times. Such changes in cell affinity are extremely important in the processes of morphogenesis. Genes and Development - Techniques and ethical issues Courtesy: Scott F. Gilbert, Michael J. F. Barresi. Developmental Biology, Sinauer Associates Oxford University Press The secrets that engage me—that sweep me away—are generally secrets of inheritance: how the pear seed becomes a pear tree, for instance, rather than a polar bear. Cynthia Ozick(1989) The future is already here. It's just not evenly distributed yet. William Gibson(1999)** Morgan redefined genetics as the science studying the transmission of traits, as opposed to embryology, the science studying the expression of traits. Within the past decade, however, the techniques of molecular biology have effected a rapprochement of embryology and genetics. Problems in animal development that could not be addressed a decade ago are now being solved by a set of techniques involving nucleic acid synthesis and hybridization. The Embryological Origins of the Gene Theory Nucleus or cytoplasm: Which controls heredity? Mendel called the genes as “form-building elements“ The gene theory that was to become the cornerstone of modern genetics originated from a controversy within the field of physiological embryology. In the late 1800s, a group of scientists began to study the mechanisms by which fertilized eggs give rise to adult organisms. Two young American embryologists, Edmund Beecher Wilson and Thomas Hunt Morgan, became part of this group of "physiological embryologists," and each became a partisan in the controversy over which of the two compartments of the fertilized egg the nucleus or the cytoplasm controls inheritance. Morgan allied himself with those embryologists who thought the control of development lay within the cytoplasm, while Wilson allied himself with Theodor Boveri, one of the biologists who felt that the nucleus contained the instructions for development. In 1896, Wilson declared that the processes of meiosis, mitosis, fertilization, and unicellular regeneration "converge to the conclusion that the chromatin is the most essential element in development." Some of the major support for the chromosomal hypothesis of inheritance was coming from the embryological studies of Theodor Boveri. Boveri fertilized sea urchin eggs with large concentrations of their sperm and obtained eggs that had been fertilized by two sperms. At first cleavage, these eggs formed four mitotic poles and divided into four cells instead of two. Boveri then separated the blastomeres and demonstrated that each cell developed abnormally, and in a different way, as aresult of each of the cells having different types of chromosomes. Thus, Boveri claimed that each chromosome had an individual nature and controlled different vital processes. In 1905, E. B. Wilson and Nettie Stevens demonstrated a critical correlation between nuclear chromosomes and organismal development: XO or XY embryos became male; XX embryos became female. The embryologist Morgan had shown that nuclear chromosomes are responsible for the development of inherited characters. Embryologists such as Frank Lillie (1927), Ross Granville Harrison (1937), Hans Spemann (1938), and Ernest E. Just (1939) claimed that there could be no genetic theory of development until at least three major challenges had been met by the geneticists: 1. Geneticists had to explain how chromosomes which were thought to be identical in every cell of the organism produce different and changing types of cell cytoplasms. 2. Geneticists had to provide evidence that genes control the early stages of embryogenesis. Almost all the genes known at the time affected the final modeling steps in development (eye color, bristle shape, wing venation in Drosophila). 3. Geneticists had to explain phenomena such as sex determination in certain invertebrates (and vertebrates such as reptiles), in which the environment determines sexual phenotype. Genomic Equivalence The other major objection to a genetically based embryology still remained: How could nuclear genes directly develop when they were the same in every cell type? Genomic equivalence is the idea that all cells in an organism have the same genetic material, or genome. This means that each cell has the information needed to create a complete organism The existence of this genomic equivalence was not so much proved as assumed (because every cell is the mitotic descendant of the fertilized egg), so one of the first problems of developmental genetics was to determine whether every cell of an organism indeed had the same set of genes, or genome, as every other cell. Metaplasia Metaplasia is a process where one type of cell is replaced by another type of cell in the same tissue. The first evidence for genomic equivalence came from embryologists studying the regeneration of excised tissues. The study of salamander eye regeneration demonstrated that even adult differentiated cells can retain their potential to produce other cell types. Therefore, the genes for these other cell types' products must still be present in the cells, though not normally expressed. In the salamander eye, removal of the neural retina promotes its regeneration from the pigmented retina, and if the lens is removed, a new lens can be formed from the cells of the dorsal iris. The regeneration of lens tissue from the iris (called Wolffian regeneration) has been intensively studied. Yamada and his colleagues found that a series of events leads to the production of a new lens from the iris. The nuclei of the dorsal side of the iris begin to synthesize enormous numbers of ribosomes, their DNA replicates, and a series of mitotic divisions ensues. The pigmented iris cells then begin to dedifferentiate by throwing out their melanosomes (the pigmented granules that give the eye its color). These melanosomes are ingested by macrophages that enter the wound site. The dorsal iris cells continue to divide, forming a globe of dedifferentiated tissue in the region of the removed lens. These cells then start synthesizing the products of differentiated lens cells, the crystallin proteins. These crystallins are made in the same order as in normal lens development. Once a new lens has formed, the cells on the dorsal side of the iris cease mitosis. Wolffian regeneration of the salamander lens from the dorsal margin of the iris. (A) Normal eye of the larval-stage newt Notophthalmus viridescens. (B-G) Regeneration of the lens, seen on days 5, 7, 9, 16, 18, and 30, respectively. The new lens is complete at day 30. (From Reyer 1954; photographs courtesy of R. W. Reyer.) Amphibian cloning: The restriction of nuclear potency The ultimate test of whether the nucleus of a differentiated cell has undergone any irreversible functional restriction is to have that nucleus generate every other type of differentiated cell in the body. If each cell's nucleus is identical to the zygote nucleus, then each cell's nucleus should be totipotent (capable of directing the entire development of the organism) when transplanted into an activated enucleated egg. Before such an experiment could be done, however, three techniques for transplanting nuclei into eggs had to be perfected: (1) a method for enucleating host eggs without destroying them; (2) a method for isolating intact donor nuclei; and (3) a method for transferring such nuclei into the host egg without damaging either the nucleus or the oocyte. All three techniques were developed in the 1950s by Robert Briggs and Thomas King. First, they combined the enucleation of the host egg with its activation. When an oocyte from the leopard frog (Rana pipiens) is pricked with a clean glass needle, the egg undergoes all the cytological and biochemical changes associated with fertilization. The internal cytoplasmic rearrangements of fertilization occur, and the completion of meiosis takes place near the animal pole of the cell. The meiotic spindle can easily be located as it pushes away the pigment granules at the animal pole, and puncturing the oocyte at this site causes the spindle and its chromosomes to flow outside the egg. The host egg is now considered both activated (the fertilization reactions necessary to initiate development have been completed) and enucleated. The transfer of a nucleus into the egg is accomplished by disrupting a donor cell and transferring the released nucleus into the oocyte through a micropipette. Some cytoplasm accompanies the nucleus to its new home, but the ratio of donor to recipient cytoplasm is only 1:105, and the donor cytoplasm does not seem to affect the outcome of the experiments. In 1952, Briggs and King, using these techniques, demonstrated that blastula cell nuclei could direct the development of complete tadpoles when transferred into the oocyte cytoplasm. Procedure for transplanting blastula nuclei into activated enucleated Rana pipiens eggs. What happens when nuclei from more advanced developmental stages are transferred into activated enucleated oocytes? King and Briggs (1956) found that whereas most blastula nuclei could produce entire tadpoles, there was a dramatic decrease in the ability of nuclei from later stages to direct development to the tadpole stage. Percentage of successful nuclear transplants as a function of the developmental age of the donor nucleus. Most somatic cells appeared to lose their ability to direct development as they became determined and differentiated. Amphibian cloning: The pluripotency of somatic cells Is it possible that some differentiatied cell nuclei differ from others in their ability to direct development? John Gurdon and his colleagues, using slightly different methods of nuclear transplantation on the frog Xenopus, obtained results suggesting that the nuclei of some differentiated cells can remain totipotent. Gurdon, too, found a progressive loss of potency with increasing developmental age, although Xenopus cells retained their potencies for a longer period than did the cells of Rana. Gurdon transferred nuclei from the intestinal endoderm of feeding Xenopus tadpoles into activated enucleated A clone of Xenopus laevis frogs. The nuclei for all the eggs. members of this clone came from a single individual—a female tailbud-stage tadpole whose parents (upper These donor nuclei contained a genetic marker (one panel) were both marked by albino genes. The nuclei (containing these defective pigmentation genes) were nucleolus per cell instead of the usual two) that transferred into activated enucleated eggs from a wild-type female (upper panel). The resulting frogs were distinguished them from host nuclei. all female and albino (lower panel). Out of 726 nuclei transferred, only 10 (1.4%) promoted development to the feeding tadpole stage. Serial transplantation (transplanting an intestinal nucleus into an egg and, when the egg had become a blastula, transferring the nuclei of the blastula cells into several more eggs) increased the yield to 7% (Gurdon 1962). In some instances, nuclei from tadpole intestinal cells were capable of generating all the cell lineages—neurons, blood cells, nerves, and so forth—of a living tadpole. Moreover, seven of these tadpoles (from two original nuclei) metamorphosed into fertile adult frogs (Gurdon and Uehlinger 1966); these two nuclei were totipotent Procedure used to obtain mature frogs from the intestinal nuclei of Xenopus tadpoles. The wild-type egg (with two nucleoli per nucleus; 2-nu) is irradiated to destroy the maternal chromosomes, and an intestinal nucleus from a marked (1-nu) tadpole is inserted. In some cases, there is no cell division; in some cases, the embryo is arrested in development; but in other cases, an entire new frog, with a 1-nu genotype, is formed. Cloning mammals In 1997, Ian Wilmut announced that a sheep had been cloned from a somatic cell nucleus from an adult female sheep. This was the first time that an adult vertebrate had been successfully cloned from another adult. To do this, Wilmut and his colleagues 1997 took cells from the mammary gland of an adult (6-year-old) pregnant ewe and put them into culture. The culture medium was formulated to keep the nuclei in these cells at the resting stage of the cell cycle (G0). They then obtained oocytes (the maturing egg cell) from a different strain of sheep and removed their nuclei. The fusion of the donor cell and the enucleated oocyte was accomplished by bringing the two cells together and sending electrical pulses through them. The electric pulses destabilized the cell membranes, allowing the cells to fuse together. Moreover, the same pulses that fused the cells activated the egg to begin development. The resulting embryos were eventually transferred into the uteri of pregnant sheep. Only 1 out of 434 sheep oocytes survived and it is DOLLY. DNA analysis confirmed that the nuclei of Dolly's cells were derived from the strain of sheep from which the donor nucleus was taken Cloned mammals, whose nuclei came from adult somatic cells. Can we clone humans unlike of other mammals? Attorney John Robertson 1998a,John Robertson 1998b has listed several reasons why cloning of humans should be legal. First, it would provide infertile couples with a chance to have a genetically related child. The government, Robertson says, should not interfere with the ability of any couple to have a genetically related child, and if cloning technologies are available, they should be used toward that end. Second, cloning could provide a source of body parts for transplantation (liver, pancreas, etc.) that would not be immunologically rejected. Third, in Robertson's view, no harm would come to the individual or society. Fourth, the techniques for cloning are not that different from other techniques of assisted reproduction, and the clone is merely a “late-born twin.” Some of the reasons cited by scientists and ethicists against Robertson's human cloning arguments are: 1. Cloning is not a good technique for giving couples a chance to have genetically related children, for scientific reasons that include the following: (a) The resulting child would be genetically related to only one member of the couple (which could cause enormous problems with divorce and custody laws). (b) With a success rate so far from 100%, a woman could not produce enough oocytes for the procedure to have a good chance of success. (c) This high failure rate predicts that many cloned fetuses would abort or be born malformed. (d) As Marie DiBerardino and Robert McKinnell, two of the pioneers of cloning, have pointed out, “In nearly 100% of the cases, [human cloning] would give rise to abnormal embryos and fetuses, almost all of which would abort. And what an atrocity if an abnormal child were born!” 2. Moral issues loom tremendous against the “spare parts” argument. A person cannot “own” another person; a clone might not wish to be used for spare parts. Scenarios that involve the creation of “brain-dead” clones kept “in storage” for spare parts are not acceptable to most of us 3. No one knows the community or personal harm in being raised by a genetically identical parent. Genetic uniqueness has always been assumed. 4. Biologically speaking, clones are not “late-born twins.” First, they fail the genetic definition of identical twins, since their mitochondrial genes (derived from the eggs of different women) would be different. Second, they fail the embryological criteria for twins in that they are not derived from the same zygote and do not share the same uterus. 5. The government already sets limits on procreation rights. Differential gene expressions Courtesy: Scott F. Gilbert, Michael J. F. Barresi. Developmental Biology, Sinauer Associates Oxford University Press