Chapter 16: Mechanisms of Genetic Variation PDF
Document Details
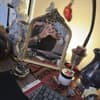
Uploaded by rafawar1000
Florida Atlantic University
Tags
Summary
This chapter details the mechanisms of genetic variation, focusing on mutations. It explains spontaneous and induced mutations, and explores different types of chemical mutagens like base analogues, DNA-modifying agents, and intercalating agents. It also examines the process of tautomerization and its role in creating mutations.
Full Transcript
16 Mechanisms of Genetic Variation Manure Happens C onsider this: A milk-producing dairy cow generates on average 150 pounds (68 kilograms) of manure each day and a beef cow will have produced about 9,800 pounds (4,445 kilograms) of manure during the finishing process that prepares it for slaughter....
16 Mechanisms of Genetic Variation Manure Happens C onsider this: A milk-producing dairy cow generates on average 150 pounds (68 kilograms) of manure each day and a beef cow will have produced about 9,800 pounds (4,445 kilograms) of manure during the finishing process that prepares it for slaughter. Multiply this by the number of dairy cows and beef cattle around the world and that’s a lot of manure! This much manure creates many problems: odor, release of volatile compounds into the atmosphere, water pollution, and the possible dissemination of pathogens. The latter is of considerable concern because it is coupled with another problem: the potential spread of antibiotic-resistant bacteria, pathogens and nonpathogens alike. Antibiotic resistance (AR) has been increasing since the beginning of antibiotic use. Currently, more than 30,000 people in Europe and the United States die each year from infections caused by microbes resistant to antimicrobial agents. You may be wondering why there might be antibiotic-resistant bacteria in manure. The answer is simple. Livestock are exposed to antibiotics either through therapeutic use or at lower amounts (subtherapeutic levels) in their feed. These antibiotics are a selective pressure that acts on the resident microbiota, leading to the evolution of antibiotic-resistant bacteria. These bacteria pass their AR genes on to their progeny. But more worrisome is their ability to share AR genes with other, unrelated bacteria in their habitat by horizontal gene transfer (HGT). Bacteria use HGT to create genetic variability, which in turn helps at least some survive when encountering a new environment (e.g., exposure to an antibiotic). Therapeutic use of antibiotics to cure infection is understandable, but what is less obvious is why livestock are given subtherapeutic levels of antibiotics in their feed. The major advantage is that feed is used more efficiently and in turn, more meat or milk is produced from each animal. This translates to greater profits for the rancher and lower costs for the consumer. Do the benefits of subtherapeutic antibiotic use outweigh the risk of spreading AR genes from manure to other organisms? Studies using molecular approaches such as those described in chapter 17 have shown the presence of AR genes in manure. Many of the genes are only distantly related to clinically relevant AR genes, and some define an entirely new family of AR genes. This is either good news or bad news. It could be that most AR genes present in manure have not spread to humans and caused disease. However, Source: Photo by Jeff Venuga/USDA Natural Resources Conservation Service it could also mean that manure is a reservoir of novel AR genes waiting to make their way into clinically important bacteria. Further complicating the issue is a recent report that manure from cows never exposed to antibiotics increases the number of soil bacteria with AR genes encoding β-lactamases (penicillin-destroying enzymes). Why this occurs is not clear. But what is clear is that bacteria are adept at altering their genomes, thereby generating more genetic diversity in a population. The increase of antibiotic resistance in bacteria is only one example of microbial evolution. All microbes use a variety of mechanisms to help ensure their survival and to evolve into new species. HGT among and between bacteria and archaea plays a particularly important role. As more and more genomes are sequenced and annotated, it is clear that HGT has occurred many times. Furthermore, genes have also been transferred from bacteria and archaea to eukaryotes and from eukaryotes to bacteria and archaea. How the genomes of microbes are altered to increase genetic diversity is the topic of this chapter. Our main focus is on how this is accomplished in the absence of sexual reproduction. Some of the mechanisms we discuss can create changes in the genome that are actually detrimental. Thus we also consider processes that help balance the stability of the genome with the capacity to introduce genetic variation. Readiness Check: Based on what you have learned previously, you should be able to: ✓ Draw a simple diagram that illustrates the three chemical moieties that define a nucleotide (section 13.2) ✓ State the base-pairing rules ✓ Explain the importance of the reading frame of a protein-coding gene (section 13.6) ✓ Describe the major types of plasmids (section 3.6) ✓ Create a concept map or table that distinguishes the secretion systems observed in Gram-negative bacteria (section 13.8) ✓ Describe the life cycle and structures of a virus (chapter 6) 375 wil11886_ch16_375-404.indd 375 23/10/18 10:18 am 376 CHAPTER 16 | Mechanisms of Genetic Variation pyrimidine for pyrimidine (e.g., a T for a C) substitutions that can eventually lead to a stable alteration of the nucleotide sequence (figure 16.1b). Such substitutions are known as transition mutations and are relatively common. On the other hand, transversion mutations—mutations where a purine is substituted for a pyrimidine (e.g., an A for a T) or a pyrimidine for a purine—are rarer due to the steric problems of pairing purines with purines and pyrimidines with pyrimidines. Replication errors can also result in the insertion and deletion of nucleotides. These mutations generally occur where there is a short stretch of repeated nucleotides. In such a location, the pairing of template and new strand can be displaced, 16.1 Mutations: Heritable Changes in a Genome After reading this section, you should be able to: a. Distinguish spontaneous from induced mutations, and list the most common ways each arises b. Construct a table, concept map, or picture to summarize how base analogues, DNA-modifying agents, and intercalating agents cause mutations c. Discuss the possible effects of mutations N N H N N Imino form of cytosine (C*) N O H N N H Enol form of thymine (T*) Adenine N N Cytosine N N N H O N N Thymine H G C T C A G O N N N N Enol form of guanine (G*) A C G T C T G C A G Wild type A C A T C T G T A G Mutant A C G T C T G C A G Wild type A C G T C T G C A G Wild type A C G T C T G T A G DNA replication A C G T C T G C A G First-generation progeny (b) N H Imino form of adenine (A*) C T G G A T H Par ental DNA Guanine H O A C T G H H Temporary enol tautomeric form of guanine DNA replication N H (a) A C G T C T G C A G N N O N N N N CH 3 H N O O H H wil11886_ch16_375-404.indd 376 N H N O N Spontaneous Mutations Spontaneous mutations result from errors in DNA replication, spontaneously occurring lesions in DNA, or the action of mobile genetic elements such as transposons (section 16.5). A few of the more prevalent mechanisms are described here. Replication errors can occur when the nitrogenous base of a nucleotide shifts to a different form (isomer) called a tautomeric form. Nitrogenous bases typically exist in the keto form (see figure 13.5) but are in equilibrium with the imino and enol forms (figure 16.1a). The shift from one form to another changes the hydrogen-bonding characteristics of the bases, allowing purine for purine (e.g., an A for a G) or H 3C H Perhaps the most obvious way genetic diversity can be created in the absence of sexual reproduction is by mutations (Latin mutare, to change), which are heritable changes in DNA sequence. Several types of mutations exist. Some arise from the alteration of single pairs of nucleotides and from the addition or deletion of one nucleotide pair in the coding regions of a gene. Such small changes in DNA are called point mutations because they affect only one base pair in a given location. Larger mutations are less common. These include large insertions, deletions, inversions, duplications, and translocations of nucleotide sequences. Mutations occur in one of two ways. (1) Spontaneous mutations arise occasionally in all cells in the absence of any added agent. (2) Induced mutations are the result of exposure to a mutagen, which can be either a physical or a chemical agent. Mutations are characterized according to either the kind of genotypic change that has occurred or their phenotypic consequences. In this section, the molecular basis of mutations and mutagenesis is first considered; then the phenotypic effects of mutations are discussed. Second-generation progeny Figure 16.1 Tautomerization and Transition Mutations. Errors in replication due to base tautomerization. (a) Normally AT and GC pairs are formed when keto groups participate in hydrogen bonds. In contrast, enol tautomers produce AC and GT base pairs. The alteration in the base is shown in blue. (b) Mutation as a consequence of tautomerization during DNA replication. The temporary enolization of guanine leads to the formation of a GT base pair in one of the first-generation progeny molecules. Thus, the pyrimidine cytosine is replaced by the pyrimidine thymine. If this abnormal pairing is not detected by repair mechanisms, when that progeny DNA molecule is replicated, one of the second-generation progeny molecules will contain an AT base pair and a GC–to–AT transition mutation will have occurred. Note that the process requires two replication cycles. Wild type is the form of the gene before mutation occurred. 23/10/18 10:18 am 16.1 Mutations: Heritable Changes in a Genome 377 (a) Slippage leading to an insertion (b) Slippage leading to a deletion 5′ C G T T T T 5′ C G T T T 3′ G C A A A A A C G T A C... 3′ G C A A A A A C G T A C... Slippage in new strand Slippage in parental strand G T 5′ C 3′ G C A A A A A C G T A C... T T T 5′ C G T T T 3′ G C A A A C G T A C... that is, to lose their base. This results in the formation of an apurinic site, which does not base pair normally and may cause a mutation after the next round of replication (figure 16.3). Likewise, pyrimidine bases (T and C) can be lost, forming an apyrimidinic site. Other lesions are caused by reactive forms of oxygen such as oxygen free radicals and peroxides produced during aerobic metabolism. For example, guanine can be converted to 8-oxo-7,8-dihydrodeoxyguanine, which often pairs with adenine, rather than cytosine, during replication. A A Induced Mutations Are Caused by Mutagens G T 5′ C 3′ G C A A A A A C G T A C... T T T T T G C A T G 5′ C G T T T G C A T G 3′ G C A A A C G T A C... A A Figure 16.2 Insertions and Deletions. A mechanism for the generation of insertions and deletions during replication. The direction of replication is indicated by the blue arrow. In each case, there is strand slippage resulting in the formation of a small loop that is stabilized by hydrogen bonding in the repetitive sequence, the AT stretch in this example. (a) If the new strand slips, an addition of one T results. (b) Slippage of the parental strand yields a deletion (in this case, a loss of two Ts). leading to insertions or deletions of bases in the new strand (figure 16.2). Spontaneous mutations can originate from lesions in DNA as well as from replication errors. For example, it is possible for purine nucleotides (A and G) to be depurinated; Any agent that damages DNA, alters its chemistry, or in some way interferes with its functioning will probably induce mutations. Mutagens are classified according to their mode of action. Three common types of chemical mutagens are base analogues, DNA-modifying agents, and intercalating agents. Some physical agents (e.g., radiation) are mutagens that damage DNA. Base analogues are structurally similar to normal nitrogenous bases and can be incorporated into the growing polynucleotide chain during replication (table 16.1). Once in place, these compounds typically exhibit base-pairing properties different from the bases they replace and can eventually cause a stable mutation. A widely used base analogue is 5-bromouracil, an analogue of thymine. It undergoes a tautomeric shift from the normal keto form to an enol much more frequently than a normal base. The enol tautomer hydrogen bonds like cytosine, pairing with guanine rather than adenine. The mechanism of action of other base analogues is similar to that of 5-bromouracil. There are many DNA-modifying agents—mutagens that change a base’s structure and therefore alter its base-pairing specificity. Some of these mutagens preferentially react with certain bases In the next round of DNA replication, the strand with the incorrect nucleotide directs synthesis of a complementary strand, thus generating a mutation. Without a proper template, the incorrect nucleotide is inserted during DNA replication. G DNA TG GC AC CG Apurinic site T GC AC CG Depurination can occur spontaneously. T GC Strand separation T GC A A CG Template strands T GC AC CG Second round of DNA Replication Strand separation First round of DNA Replication TG GC AC CG Wild-type sequence Mutant T GC A GCG A A CG T T GC A A CG The strand with the apurinic site can continue to direct synthesis of complementary strands having an incorrect nucleotide. Figure 16.3 Mutation Due to Formation of an Apurinic Site. Apurinic sites arise spontaneously (depurination). They do not provide a template for DNA replication, and as a result, an incorrect nucleotide can be inserted. A similar process introduces mutations at sites where apyrimidinic sites arise. wil11886_ch16_375-404.indd 377 23/10/18 10:18 am 378 CHAPTER 16 Table 16.1 | Mechanisms of Genetic Variation N O Examples of Mutagens Pairs normally with cytosine H N H N H N Guanine N H CH3 O N H N C N NH NO2 N-methyl-N′-nitro-N-nitrosoguanidine CH3 N O Sometimes pairs with thymine H O6- methylguanine N N N H N H Figure 16.4 Methyl-Nitrosoguanidine Mutagenesis. Methylnitrosoguanidine methylates guanine. and produce a particular kind of DNA damage. For example, methylnitrosoguanidine adds methyl groups to guanine, causing it to mispair with thymine (figure 16.4). A subsequent round of replication can then result in a GC-AT transition. Intercalating agents distort DNA to induce single nucleotide pair insertions and deletions. These mutagens are planar and insert themselves (intercalate) between the stacked bases of the helix. This results in distortion of the helical structure, rendering H O O P O CH2 O– H H N H O H Thymine O CH2 O– H H H H H CH2 H N O Thymine H N O O O H H N H CH3 H H O O N O Ultraviolet light O H P O– CH3 CH3 O P O O H H O N O H O the DNA inactive as a template for either replication or transcription. Many mutagens, and indeed many carcinogens, damage bases so severely that hydrogen bonding between base pairs is impaired or prevented and the damaged DNA can no longer act as a template for replication. For instance, ultraviolet (UV) radiation often generates thymine dimers between adjacent thymines (figure 16.5). O P O CH2 O– H H CH3 H O O H H N H N H O Thymine dimer Figure 16.5 Thymine Dimers in a DNA Strand Are Formed by Ultraviolet Radiation. Thymine dimers are one type of cyclobutane dimer (i.e., a dimer formed between adjacent pyrimidines) generated by UV radiation. wil11886_ch16_375-404.indd 378 23/10/18 10:18 am 16.1 Mutations: Heritable Changes in a Genome 379 Effects of Mutations The effects of mutations can be described at the protein level and in terms of observed phenotypes. In all cases, the impact is readily noticed only if it produces a change in phenotype. In Table 16.2 general, the more prevalent form of a gene and its associated phenotype is called the wild type. A change from wild type to a mutant form is a forward mutation (table 16.2). It is possible to restore a wild-type phenotype with a second mutation. If the Types of Point Mutations STOP! Ala Arg Cys Pro Ala wil11886_ch16_375-404.indd 379 Glu Arg 23/10/18 10:18 am 380 CHAPTER 16 | Mechanisms of Genetic Variation second mutation is at the same site as the original mutation (e.g., the same base pair in a codon), it is called a reversion mutation. Some reversion mutations re-establish the original wild-type sequence. Others create a new codon that codes for the same amino acid. Reversions can also restore the wild-type phenotype by creating a codon that replaces the wild-type amino acid with a similar amino acid (e.g., both amino acids are nonpolar). If the wild-type phenotype is restored by a second mutation at a different site than the original mutation, it is called a suppressor mutation. Suppressor mutations may be within the same gene (intragenic suppressor mutation) or in a different gene (extragenic suppressor mutation). Because point mutations are the most common types of mutations, their effects are our focus. Mutations in Protein-Coding Genes Point mutations in protein-coding genes affect protein structure in a variety of ways. Point mutations are named according to if and how they change the encoded protein. The most common types of point mutations are silent mutations, missense mutations, nonsense mutations, and frameshift mutations. Examples of each are shown in table 16.2. Silent mutations change the nucleotide sequence of a codon but do not change the amino acid encoded by that codon. This is possible because the genetic code exhibits degeneracy. Therefore when there is more than one codon for a given amino acid, a single base substitution may result in the formation of a new codon for the same amino acid. For example, if the codon CGU were changed to CGC, it would still code for arginine, even though a mutation had occurred. When there is no change in the protein, there is no change in the phenotype of the organism. The genetic code consists of three-letter “words” (section 13.6) Missense mutations involve a single base substitution that changes a codon for one amino acid into a codon for another. For example, the codon GAG, which specifies glutamic acid, could be changed to GUG, which codes for valine. The effects of missense mutations vary. They alter the primary structure of a protein, but the effect of this change may range from complete loss of activity to no change at all. This is because the effect of missense mutations on protein function depends on the type and location of the amino acid substitution. For instance, replacement of a nonpolar amino acid in the protein’s interior with a polar amino acid can drastically alter the protein’s three-dimensional structure and therefore its function. Similarly the replacement of a critical amino acid at the active site of an enzyme often destroys its activity. However, the replacement of one polar amino acid with another at the protein surface may have little or no effect. Such mutations are called neutral mutations. Missense mutations play a very important role in providing new variability to drive evolution because they often are not lethal and therefore remain in the gene pool. Proteins (appendix I) Mutation by Base Substitution wil11886_ch16_375-404.indd 380 Nonsense mutations convert a sense codon (i.e., one that codes for an amino acid) to a nonsense codon (i.e., a stop codon: one that does not code for an amino acid). This causes the early termination of translation and therefore results in a shortened polypeptide. Depending on the location of the mutation, the phenotype may be more or less severely affected. Most proteins retain some function if they are shortened by only a few amino acids; complete loss of normal function usually results if the mutation occurs closer to the beginning or middle of the gene. Frameshift mutations arise from the insertion or deletion of base pairs within the coding region of the gene. Since the code consists of a precise sequence of triplet codons, the addition or deletion of fewer than three base pairs causes the reading frame to be shifted for all subsequent codons. Frameshift mutations usually are very deleterious and yield mutant phenotypes resulting from the synthesis of nonfunctional proteins. In addition, stop codons often occur downstream of frameshift mutations so that the peptide product is shorter as well as different in sequence. Of course, if the frameshift occurs near the end of the gene or if there is a second frameshift shortly downstream from the first that restores the reading frame, the phenotypic effect might not be as drastic. A second, nearby frameshift that restores the proper reading frame is an example of an intragenic suppressor mutation (table 16.2). Addition and Deletion Mutations Changes in protein structure can alter the phenotype of an organism in many ways. They may change the microorganism’s colony or cellular morphology. Lethal mutations, when expressed, result in the death of the microorganism. Because a microbe must be able to grow to be isolated and studied, lethal mutations are recovered only if they are conditional mutations. Conditional mutations are those that are expressed only under certain environmental conditions. For example, a conditional lethal mutation might not be expressed when a bacterium is c ultured at a low temperature but would be expressed at a high temperature. Thus the mutant would grow normally at cooler temperatures but would die at high temperatures. Other common mutations inactivate a biosynthetic pathway, frequently eliminating the capacity of the mutant to make an essential molecule such as an amino acid or nucleotide. A strain bearing such a mutation has a conditional phenotype: It is unable to grow on medium lacking that molecule but grows when the molecule is provided. Such mutants are called auxotrophs, and they are said to be auxotrophic for the molecule they cannot synthesize. The wild-type strain from which the mutant arose is called a prototroph. Another interesting mutant is the resistance mutant. These mutants have acquired resistance to some pathogen (e.g., bacteriophage), chemical (e.g., antibiotic), or physical agent. Auxotrophic and resistance mutants are quite important in microbial genetics due to the ease of their detection and their relative abundance. 23/10/18 10:18 am 16.2 Detection and Isolation of Mutants 381 Mutations in Regulatory Sequences Some of the most interesting and informative mutations studied by microbial geneticists are those that occur in the regulatory sequences responsible for controlling gene expression. Constitutive lactose operon mutants in E. coli are excellent examples. Many of these mutations map in the operator site and produce altered operator sequences that are not recognized by the lac repressor protein. Therefore the operon is continuously transcribed, and β-galactosidase is always synthesized. Mutations in promoters also have been identified. If the mutation renders the promoter sequence nonfunctional, the mutant will be unable to synthesize the product, even though the coding region of the structural gene is completely normal. Without a fully functional promoter, RNA polymerase cannot transcribe a gene as well as wild type. Regulation of transcription initiation saves considerable energy and materials (section 14.2) Because mutations provide genetic diversity, which enhances survival during changing environmental conditions, mutations are of value to microbes. Mutations are also of practical importance to microbial geneticists. Mutant strains have been used to reveal mechanisms of complex processes such as DNA replication, endospore formation, and regulation of transcription. They are also useful as selective markers in recombinant DNA procedures. Microbial DNA technologies (chapter 17) To study microbial mutants, they must be readily detected, even when they are rare, and then efficiently isolated from wild-type organisms and other mutants not of interest. Microbial geneticists typically increase the likelihood of obtaining mutants by using mutagens to increase the rate of mutation. The rate can increase from the usual one mutant per 107 to 1011 cells to about one per 103 to 106 cells. Even at this rate, carefully devised methods for detecting or selecting a desired mutation must be used. This section describes some techniques used in mutant detection, selection, and isolation. Mutations in tRNA and rRNA Genes Mutations in tRNA and rRNA alter the phenotype of an organism through disruption of protein synthesis. In fact, these mutants often are initially identified because of their slow growth. On the other hand, a suppressor mutation involving tRNA restores normal (or near normal) growth rates. In these mutations, a base substitution in the anticodon region of a tRNA allows the insertion of the correct amino acid at a mutant codon (table 16.2). Comprehension Check 1. List three ways in which spontaneous mutations might arise. 2. Compare and contrast the means by which the mutagens 5-bromouracil, methyl-nitrosoguanidine, proflavin, and UV radiation induce mutations. 3. Give examples of intragenic and extragenic suppressor mutations. 4. Sometimes a point mutation does not change the phenotype. List all the reasons why this is so. 5. Why might a missense mutation at a protein’s surface not affect the phenotype of an organism, whereas the substitution of an internal amino acid does? 16.2 Detection and Isolation of Mutants After reading this section, you should be able to: a. Differentiate mutant detection from mutant selection b. Design an experiment to isolate mutant bacteria that are auxotrophic for a specific amino acid c. Propose an experiment to isolate revertants of an amino acid auxotroph and predict the types of mutations that might lead to the revertant phenotype d. Explain how the Ames test is used to screen for potential carcinogens and evaluate its effectiveness wil11886_ch16_375-404.indd 381 Mutant Detection: Screening for Mutants with an Observable Phenotype When collecting mutants of a particular organism, the wild-type characteristics must be known so that an altered phenotype can be recognized. A suitable detection system for the mutant phenotype also is needed. The use of detection systems is called screening. Screening for mutant phenotypes in haploid organisms is straightforward because the effects of most mutations can be seen immediately as long as the mutated gene is expressed under the growth conditions used. Some screening procedures require only examination of colony morphology. For instance, if colorless-colony mutants of a bacterium that normally produces red colonies are sought, detection simply requires visual observation of colony color. Other screening methods are more complex. For example, the replica plating technique is used to screen for auxotrophic mutants. It distinguishes between mutants and the wild-type strain based on their ability to grow in the absence of a particular biosynthetic end product (figure 16.6). A lysine auxotroph, for instance, grows on lysine-supplemented media but when the colonies that grow on this medium are transferred to a medium lacking lysine, they will not grow, because they cannot synthesize this amino acid. Once a screening method is devised, mutants are collected. However, mutant collection can present practical problems. Consider a search for the colorless-colony mutants mentioned previously. If the mutation rate were around one in a million, on average a million or more organisms would have to be tested to find one colorless-colony mutant. This probably would require several thousand plates. The task of isolating auxotrophic mutants in this way would be even more taxing with the added labor of replica plating. Thus, if possible, it is more efficient to use a selection system employing some environmental factor to inhibit the growth of wild-type microorganisms. Examples of selection systems are described next. 23/10/18 10:18 am 382 CHAPTER 16 | Mechanisms of Genetic Variation Mutant Selection: Using Conditions to Inhibit Growth of Wild-Type Organisms Treatment of E. coli cells with a mutagen, such as nitrosoguanidine Replica block Velvet surface (sterilized) Master plate (complete medium) Inoculate a plate containing complete growth medium and incubate. Both wild-type and mutant survivors form colonies. Replica plate (complete medium) Replica plate (medium minus lysine) Incubation All strains grow. No growth of lysine auxotroph Culture lysine _ auxotroph (Lys ) (medium with lysine) Figure 16.6 Replica Plating. The use of replica plating to isolate a lysine auxotroph. After growth of a mutagenized culture on a complete medium, a piece of sterile velvet is pressed on the plate surface to pick up bacteria from each colony. Then the velvet is pressed to the surface of other plates, and organisms are transferred to the same position as on the master plate. After the location of Lys− colonies growing on the replica with complete medium is determined, the auxotrophs can be isolated and cultured. MICRO INQUIRY How would you screen for a tryptophan auxotroph? How would you select for a mutant that is resistant to the antibiotic ampicillin but sensitive to tetracycline (assume the parental stain is resistant to both antibiotics)? wil11886_ch16_375-404.indd 382 Mutant selection techniques use incubation conditions under which the mutant grows because of properties conferred by the mutation, whereas the wild type does not. Selection methods often involve reversion or suppressor mutations that restore the wild-type phenotype. For example, if the intent is to isolate a prototroph from a lysine auxotroph (Lys−), a large population of lysine auxotrophs is plated on minimal medium lacking lysine, incubated, and examined for colony formation. Only cells that have mutated to restore the ability to manufacture lysine (i.e., prototrophy) will grow on minimal medium. Several million cells can be plated on a single Petri dish, but only the rare revertant cells will grow. Thus many cells can be tested for mutations by scanning a few Petri dishes for growth. Other selection methods identify mutants resistant to a particular environmental stress such as virus attack, antibiotic treatment, or specific temperatures. Therefore it is possible to grow the microbe in the presence of the stress and look for surviving organisms. Consider the example of an antibioticsensitive wild-type bacterium. When it is cultured in medium lacking the antibiotic and then plated on selective medium containing the antibiotic, any colonies that form are resistant to the antibiotic and carry a mutated gene that confers antibiotic resistance. Mutant screening and selection methods are used for purposes other than understanding more about the nature of genes or the biochemistry of a particular microorganism. One very important role of mutant selection and screening techniques is in the study of carcinogens. One of the first and perhaps best known of the carcinogen testing systems is the Ames Test, developed by Bruce Ames. The Ames test is based on the observation that many carcinogens also are mutagens. The test determines if a substance increases the rate of mutation; that is, if it is a mutagen. If the substance is a mutagen, then it is also likely to be carcinogenic if an animal is exposed to it at sufficient levels. Note that the test does not directly test for carcinogenicity. This is because it uses a bacterium as the test organism. Carcinogenicity can only be directly demonstrated with animals, and testing is extremely expensive and takes much longer to complete than does the Ames test. Thus the Ames test serves as an inexpensive procedure to identify chemicals that may be carcinogenic and thus deserve further testing. The Ames test is called a mutational reversion assay because it looks for histidine prototrophs that arise from histidine auxotrophs. The assay employs several “tester” strains of Salmonella enterica serovar Typhimurium. Each tester strain has a different mutation in the histidine biosynthesis operon. They also have mutations that make their cell walls more permeable to test substances and impair their ability to repair DNA. In the Ames test, tester strains of S. Typhimurium are plated with the substance being tested and the number of visible colonies that form is determined (figure 16.7). To ensure that DNA 23/10/18 10:18 am 16.3 DNA Repair Maintains Genome Stability 383 Culture of Salmonella histidine auxotrophs Plate culture Minimal medium plus a small amount of histidine Minimal medium with test mutagen and a small amount of histidine Incubate at 37°C Spontaneous revertants Revertants induced by the mutagen Figure 16.7 The Ames Test for Mutagenicity. MICRO INQUIRY Why is a small amount of histidine added to the test medium? replication can take place and allow for the development of a mutation in the presence of the potential mutagen, the bacteria and test substance are mixed in dilute molten top agar to which a trace of histidine has been added. This molten mix is then poured on top of minimal agar plates and incubated for 2 to 3 days at 37°C. All of the histidine auxotrophs grow for the first few hours in the presence of the test compound until the histidine is depleted. This initial growth does not produce a visible colony. Once the histidine supply is exhausted, only revertants that have mutated to regain the ability to synthesize histidine continue to grow and produce visible colonies. These colonies need only be counted and compared to controls to estimate the relative mutagenicity of the compound: The more colonies, the greater the mutagenicity. Comprehension Check 1. Describe how replica plating is used to detect and isolate auxotrophic mutants. 2. Why are mutant selection techniques generally preferable to screening methods? 3. Briefly discuss how reversion mutations and resistance to an environmental factor (e.g., presence of an antibiotic) can be employed in mutant selection. 4. Describe how you would isolate a mutant that required histidine for growth and was resistant to penicillin. The wild type is a prototroph. 5. What is the Ames test and how is it carried out? What assumption concerning mutagenicity and carcinogenicity is it based upon? wil11886_ch16_375-404.indd 383 16.3 DNA Repair Maintains Genome Stability After reading this section, you should be able to: a. Compare and contrast proofreading, excision repair, direct repair, mismatch repair, and recombinational repair b. Propose a scenario that would elicit the SOS response and describe the response to those conditions If there is a microbial equivalent of an extreme sport, Deinococcus radiodurans’s ability to repair its genome after it has been blasted apart by a high dose of radiation might be a contender. Surprisingly, this ability is primarily related to the resistance of D. radiodurans proteins and the structure of its genome, rather than to its DNA repair mechanisms. Following damage, its radiation-resistant proteins begin repairing the genome quickly. Repair is aided by the genome c onsisting of two chromosomes, each having numerous areas of homology. D. radiodurans is polyploid, that is, there are several copies of the chromosome. This allows the DNA fragments to anneal, allowing the shattered genome to be pieced together. Other than this, D. radiodurans uses pretty much the same DNA repair mechanisms as other organisms. Obviously mutations can have disastrous effects. Therefore it is imperative that a microorganism be able to repair changes. There are numerous repair mechanisms and these are well conserved across all domains of life. Repair in E. coli is best understood and is our focus in this section. DeinococcusThermus includes radiation-resistant bacteria (section 21.2) Proofreading: The First Line of Defense As we discuss in chapter 13, replicative DNA polymerases sometimes insert the incorrect nucleotide during DNA replication. However, these DNA polymerases have the ability to evaluate the hydrogen bonds formed between the newly added nucleotide and the template nucleotide, and correct any errors immediately; that is, before the next nucleotide is added. This ability is called proofreading. When a DNA polymerase detects that a mistake has been made, it backs up and removes the incorrect nucleotide with its 3 ′ to 5́ ′ exonuclease activity (see figure 13.16). It then restarts DNA replication, this time inserting the correct nucleotide. Proofreading is efficient, but does not correct every error. Furthermore, it does not correct induced mutations. E. coli thus uses other repair mechanisms to help ensure the stability of its genome. DNA replication in bacteria (section 13.3) Mismatch Repair When proofreading by replicative DNA polymerases fails, mismatched bases are usually detected and repaired by the mismatch repair system (figure 16.8). In E. coli the enzyme MutS scans newly replicated DNA for mismatched pairs. Another enzyme, MutH, removes a stretch of newly synthesized DNA around the 23/10/18 10:18 am 384 CHAPTER 16 | Mechanisms of Genetic Variation The MutS protein slides along the DNA until it finds a mismatch. MutL binds MutS and the MutS/MutL complex binds to MutH, which is already bound to a hemimethylated sequence. m GATC CTAG Parental strand MutH Newly made strand newly synthesized DNA strands temporarily lack methyl groups on their bases, whereas the parental DNA is methylated. Thus for a short time after the replication fork has passed, the DNA is hemimethylated, that is, methylated only on one strand. The repair system cuts out the mismatch from the unmethylated strand. Eventually adenine bases in the GATC sequences of the newly synthesized strand are methylated by enzyme DNA adenine methyltransferase (Dam). MutL MutS T G Incorrect base MutH makes a cut in the nonmethylated strand. An exonuclease begins at this cleavage site and then digests the nonmethylated strand just beyond the base mismatch. m GATC MutH cleavage site G DNA polymerase fills in the vacant region. DNA ligase seals the ends. m GATC CTAG Excision repair corrects damage that causes distortions in the DNA double helix. Two types of excision repair systems have been described: nucleotide excision repair and base excision repair. Both use the same approach to repair; they remove the damaged portion of a DNA strand and use the intact complementary strand as the template for synthesis of new DNA. The two repair systems are distinguished by the enzymes used to correct the damage. In nucleotide excision repair, an E. coli enzyme called UvrABC endonuclease removes damaged nucleotides and a few nucleotides on either side of the lesion. The resulting single-stranded gap is filled by DNA polymerase I, and DNA ligase joins the fragments (figure 16.9). This system can remove thymine dimers (figure 16.5) and repair almost any other injury that produces a detectable distortion in DNA. Base excision repair employs enzymes called DNA glycosylases. These enzymes remove damaged or unnatural bases yielding apurinic or apyrimidinic (AP) sites. Enzymes called AP endonucleases recognize the damaged DNA and nick the backbone at the AP site (figure 16.10). DNA polymerase I removes the damaged region, using its 5 ′ to 3 ′ exonuclease activity. It then fills in the gap, and DNA ligase joins the DNA fragments. Direct Repair The mismatch has been repaired correctly. C G Figure 16.8 Methyl-Directed Mismatch Repair in E. coli. The role of MutH is to identify the methylated strand of DNA, which is the nonmutated parental strand. The methylated adenine is designated by an m. MICRO INQUIRY How is mismatch repair similar to DNA polymerase proofreading? How is it different? mismatch. A DNA polymerase then replaces the excised nucleotides, and the resulting nick is sealed by DNA ligase. Successful mismatch repair depends on the ability of mismatch repair enzymes to distinguish between parental and newly synthesized DNA strands. This distinction is possible because wil11886_ch16_375-404.indd 384 Excision Repair Thymine dimers and alkylated bases (e.g., those with methyl or ethyl groups attached) often are corrected by direct repair. Thymine dimers (figure 16.5) are split apart by a process called photoreactivation, which requires visible light and is catalyzed by the enzyme photolyase. Methyl and some other alkyl groups that have been added to guanine can be removed with the help of an enzyme known as alkyltransferase or methylguanine methyltransferase. Thus damage to guanine from mutagens such as methyl-nitrosoguanidine (figure 16.4) can be repaired directly. Direct Repair Recombinational Repair Recombinational repair corrects damaged DNA in which both bases of a pair are missing or damaged, or where there is a gap opposite a lesion. In this type of repair, the protein RecA cuts a piece of template DNA from a sister molecule and puts it into the gap or uses it to replace a damaged strand (figure 16.11). Another copy of the damaged segment often is available because either it has recently been replicated or the cell contains more than one copy of its chromosome. Once the template is in place, the remaining damage can be corrected by another repair system. 23/10/18 10:18 am 16.3 DNA Repair Maintains Genome Stability 385 5′ Thymine dimer 5′ 3′ A 3′ T T A 5′ B 3′ 5′ 3′ A 3′ T T 5′ 3′ G C T C A A C G A C G A C C T G C T G C T G G A C G A G T A 5′ B DNA glycosylase recognizes an abnormal base and cleaves the bond between the base and the sugar. U The UvrAB complex tracks along the DNA in search of damaged DNA. 5′ 3′ G C T C A A C G A C G A C C T C G A G T U G C T G C T G G A 3′ After damage is detected, UvrA is released and UvrC binds. 5′ 5′ Apyrimidinic nucleotide AP endonuclease recognizes a missing base and cleaves the DNA backbone on the 5′ side of the missing base. 3′ T T 3′ 5′ 5′ B Uvr C 3′ UvrC makes cuts on both sides of the thymine dimer. Cut Cut 3′ T T B Uvr C DNA polymerase uses its 5′ 3′ exonuclease activity to remove the damaged region and then fills in the region with normal DNA. DNA ligase seals the region. 5′ 3′ T C C C C C T G C A A G A G A A G G G G G A G C T T C T C T 5′ 3′ UvrD is a helicase that removes the damaged region. UvrB and UvrC are also released. 5′ Figure 16.10 Base Excision Repair. 5′ 3′ 3′ 5′ DNA polymerase fills in the gap and DNA ligase joins the fragments. No thymine dimer 5′ 3′ 3′ 5′ Figure 16.9 Nucleotide Excision Repair in E. coli. MICRO INQUIRY How is damaged DNA recognized by the UvrAB complex? SOS Response Despite having multiple repair systems, sometimes the damage to an organism’s DNA is so great that the normal repair mechanisms just described cannot repair all the damage. As a result, DNA synthesis stops completely. In such situations, a global control network called the SOS response is activated. In this wil11886_ch16_375-404.indd 385 5′ Nick 5′ 3′ 3′ G C T C A A C G A C G A C C T G C T G C T G G A C G A G T response, over 50 genes are activated when a transcriptional repressor protein called LexA is destroyed. The SOS response, like recombinational repair, depends on the activity of RecA. RecA binds to single- or double-stranded DNA breaks and gaps generated by cessation of DNA synthesis. RecA binding initiates recombinational repair. Simultaneously RecA takes on coprotease function, causing LexA to destroy itself (autoproteolysis). The first genes transcribed in the SOS response encode the Uvr proteins needed for nucleotide excision repair (figure 16.9). Then expression of genes whose products are involved in recombinational repair is increased. To give the cell time to repair its DNA, the protein SfiA is produced; SfiA blocks cell division. Finally, if the DNA has not been fully repaired after about 40 minutes, a process called translesion DNA synthesis is triggered. In this process, DNA polymerase IV (also known as DinB; Din is short for damage inducible) and DNA polymerase V (also called UmuCD; Umu is short for UV mutagenesis) synthesize DNA across gaps and other lesions (e.g., thymine dimers) that stopped DNA polymerase III. However, because an intact template does not exist, these DNA polymerases often insert incorrect bases. Furthermore, they lack proofreading activity. Therefore even though DNA synthesis continues, it is highly error prone and results in the generation of numerous mutations. 23/10/18 10:18 am 386 CHAPTER 16 | Mechanisms of Genetic Variation The SOS response is so named because it is made in a life-ordeath situation. The response increases the likelihood that some cells will survive by allowing DNA synthesis to continue. For the cell, the risk of dying because of failure to replicate DNA is greater than the risk posed by the mutations generated by this error-prone process. A B Comprehension Check Gap T T Thymine dimer C D By recombination, a region in strand A is swapped for the same region in strand C. Swapped region 1. Compare and contrast the two types of excision repair. 2. What role does DNA methylation play in mismatch repair? 3. When E. coli cells are growing rapidly, they may contain up to four copies of their chromosomes. Why is this important if E. coli needs to carry out recombinational repair? 4. Explain how the following DNA alterations and replication errors would be corrected (there may be more than one way): base addition errors by DNA polymerase III during replication, thymine dimers, AP sites, methylated guanines, and gaps produced during replication. 16.4 Microbes Use Mechanisms Other than Mutation to Create Genetic Variability After reading this section, you should be able to: a. Distinguish vertical gene transfer from horizontal gene transfer b. Summarize the four possible outcomes of horizontal gene transfer c. Compare and contrast homologous recombination and site-specific recombination Swapped region T T The gap that is now in strand A is filled in by DNA polymerase and DNA ligase, using strand B as a template. Repaired gap T T The gap has been repaired, but the thymine dimer remains. It will be removed by another repair system. Figure 16.11 Recombinational Repair. In section 16.1, we discuss the consequences of mutations in terms of their effect on a protein and the phenotype of the organisms bearing the mutation. However, the effect also depends on the environment in which the organism lives. In other words, those mutations that don’t immediately kill the organism are subject to selective pressure. Selective pressure determines if a mutation will persist in a population to become an alternate form of the gene. An alternate form of a gene is termed an allele. The existence of alleles for each gene in a genome means each organism in a population can have a distinctive (and probably a unique) set of alleles making up its genome, that is, its genotype. Each genotype in a population can be selected for or selected against. Organisms with genotypes, and therefore phenotypes, best suited to the environment survive and are most likely to pass on their genes. Shifts in environmental pressures lead to changes in the population and ultimately result in the evolution of new species. The mechanisms by which new combinations of genes are generated are the topic of this section. All involve recombination, the process in which one or more nucleic acid molecules are rearranged or combined to produce a new genotype. Geneticists refer to organisms produced following a recombination event as recombinant organisms or simply recombinants. Sexual Reproduction and Genetic Variability The transfer of genes from parents to progeny is called vertical gene transfer. This type of gene transfer is observed in all wil11886_ch16_375-404.indd 386 23/10/18 10:18 am 16.4 Microbes Use Mechanisms Other than Mutation to Create Genetic Variability 387 organisms. In eukaryotes capable of sexual reproduction, vertical gene transfer is accompanied by genetic recombination, which occurs in two ways. The first is from crossing-over between sister chromosomes during meiosis. This creates new mixtures of alleles on homologous chromosomes. The second type of recombination results from fusion of gametes. Since each gamete contains alleles from the parent, when the gametes fuse, the parental alleles are combined in the zygote. As a result of these two types of recombination events, progeny organisms are not identical to their parents or to each other. Horizontal Gene Transfer: Creating Variability the Asexual Way Bacteria and archaea do not reproduce sexually. This suggests that genetic variation in populations of these microbes should be relatively limited, only occurring with the advent of a new mutation and its passage to the next generation by vertical gene transfer. However, this is not the case. Bacteria and archaea have multiple mechanisms for creating recombinants collectively referred to as horizontal (lateral) gene transfer (HGT). HGT is distinctive from vertical gene transfer because genes from one independent, mature organism are transferred to another mature organism, often creating a stable recombinant having characteristics of both the donor and the recipient. Horizontal Gene Transfer The importance of HGT cannot be overstated. HGT has been important in the evolution of many species, and it continues to be commonplace in many environments. Indeed, HGT is the main mechanism by which bacteria and archaea evolve. Many examples are known of DNA transfer between distantly related genera and across domains of life, particularly among microbes sharing a habitat (e.g., the human gut or an Antarctic lake). HGT helps bacteria and archaea survive environmental stresses. For instance, some archaea respond to UV radiation by increasing their ability to carry out HGT so that they can obtain and use DNA from other cells to repair any UV-damaged DNA in their genomes. Another important example is the transfer of groups of genes encoding virulence factors from pathogens to other bacteria. These gene clusters are called pathogenicity islands, and their discovery has provided valuable insights into the evolution of pathogenic bacteria. Finally, in most cases, the acquisition of new traits by HGT enables microbes to rapidly expand their ecological niche. This is clearly seen when HGT results in the spread of antibiotic-resistance genes among pathogenic bacteria. Evolutionary processes and the concept of a microbial species inspire debate (section 19.5); Pathogenicity islands encode virulence factors (section 35.4) During HGT, a piece of donor DNA, sometimes called the exogenote, enters a recipient cell. The transfer can occur in multiple ways. If the donor and recipient cells are closely related, the DNA may be transferred directly between the two cells in temporary physical contact (conjugation). Alternately, a bacteriophage may act as an intermediary, and transport DNA within the viral capsid (transduction). In both cases, cell surface structures dictate the range of organisms that may interact by these mechanisms. Two other ways that HGT occurs are through transfer of naked DNA (transformation) or transfer of DNA within a membrane vesicle. In these processes, the DNA is taken up directly from the environment, and the donor and recipient may not be closely related. All of these processes have been described in bacteria and archaea. In addition, membrane vesicle formation occurs in cells from all domains, and although the phenomenon of HGT by membrane vesicles is well documented, the mechanistic details remain obscure. Thus we focus on the well-established HGT processes of conjugation, transformation, and transduction. Membrane vesicles are an alternative secretion mechanism (section 13.8) If the donor DNA contains genes already present in the recipient, the recipient will become temporarily diploid for those genes. This partially diploid cell is sometimes called a merozygote (Greek meros, part). The donor DNA has four possible fates in the recipient (figure 16.12). First, when the Repr oduc Integration of donor DNA Donor DNA Partially diploid recipient cell Conjugation Transformation Transduction Membrane Vesicles Recipient chromosome tion tion oduc Repr s eplicate NA self-r Donor D lasmid). (e.g., a p Dono Recombinant r cell self-r DNA can n e o p Re t licate Recip. de stri gr ctio repro ient ad n du ati or dono ces; on C r R does DNA of IS not. do PR no /C r D as NA ion uct rod p e R Population of stable recombinants No stable recombinants Figure 16.12 The Fate of Donor DNA During Horizontal Gene Transfer. wil11886_ch16_375-404.indd 387 23/10/18 10:18 am 388 CHAPTER 16 | Mechanisms of Genetic Variation donor DNA has a sequence homologous to one in the recipient’s chromosome (sometimes called an endogenote), integration may occur. That is, the donor’s DNA may pair with the recipient’s DNA and recombine. The recombinant then reproduces, yielding a population of stable genetic variants. Second, if the donor DNA is able to replicate (e.g., it is a plasmid), it may persist separate from the recipient’s chromosome. When the recipient reproduces, the donor DNA replicates and a population of stable recombinants is formed. Third, the donor DNA remains in the cytoplasm but is unable to replicate. When the recipient divides, the donor DNA is eventually lost from the population. Finally, host restriction or CRISPR/Cas degradation of donor DNA may occur, thereby preventing the formation of a recombinant cell. Membrane vesicles are an alternative secretion mechanism (section 13.8); Responses to viral infection (section 14.6) Molecular Recombination: Joining DNA Molecules Together Crossing-over during meiosis and integration of donor DNA into the recipient’s chromosome during HGT occur by similar mechanisms. Homologous recombination is the most common mechanism, and many of the enzymes used are similar for all organisms. Homologous recombination occurs wherever there are long regions of the same or similar nucleotide sequence in two DNA molecules (e.g., sister chromosomes in diploid eukaryotes or similar sequences on both a chromosome and plasmid). Homologous recombination results from DNA strand breakage and reunion leading to crossing-over. It is carried out by enzymes, many of which are also important for DNA repair (e.g., RecA; section 16.3). The other major type of recombination is site-specific recombination. Site-specific recombination differs from homologous recombination in three significant ways. First, it does not require long regions of sequence homology. Second, recombination occurs at specific target sites in DNA molecules, and third, it is catalyzed by enzymes collectively called recombinases. Site-specific recombination is used by some plasmids and viral genomes to integrate into host chromosomes. It is also the type of recombination used by transposable elements to move from one place to another in DNA molecules, as we discuss in section 16.5. Comprehension Check 1. An antibiotic-resistance gene located on a bacterium’s chromosome is transferred from the bacterium to its progeny. What type of gene transfer is this? 2. What four fates can DNA have after entering a bacterium? 3. How does homologous recombination differ from site-specific recombination? wil11886_ch16_375-404.indd 388 16.5 Transposable Elements Move Genes Within and Between DNA Molecules After reading this section, you should be able to: a. Differentiate insertion sequences from transposons b. Distinguish simple transposition from replicative transposition c. Defend this statement: “Transposable elements are important factors in the evolution of bacteria and archaea” As more and more genomes are sequenced and annotated, it is apparent that all genomes are rife with genetic elements that are able to move into and out of the genomes where they reside. These elements are often referred to as “jumping genes,” mobile genetic elements, and transposable elements. Transposition refers to the movement of a mobile genetic element. Mobile genetic elements were first discovered in the 1940s by Barbara McClintock during her studies on maize genetics (a discovery for which she was awarded the Nobel Prize in 1983). As additional types of mobile genetic elements were discovered, nomenclature was developed to distinguish them. As is often the case when a scientific field expands and its language evolves, the nomenclature became unwieldy and confusing. In 2008 a group of scientists studying bacterial and archaeal mobile genetic elements proposed a new definition for transposable elements: “specific DNA segments that can repeatedly insert into one or more sites or into one or more genomes.” This new definition encompasses elements that differ in structure, mechanisms of integration and excision, target sites, and ability to be transferred from one cell to another by HGT. Transposition: Shifting Segments of the Genome The enzymes that function in transposition are collectively termed recombinases. However, the recombinase used by a specific mobile genetic element may be called an integrase, resolvase, or transposase. There is tremendous interest in these enzymes because their mechanisms of action are similar to those used to rearrange the gene segments that encode important immune system proteins such as antibodies and T-cell receptors. Adaptive immunity (chapter 33) The simplest mobile genetic elements in bacteria are insertion sequences, or IS elements for short (figure 16.13a). An IS element is a short sequence of DNA (around 750 to 1,600 base pairs [bp] in length). It contains only the gene for the enzyme transposase, and it is bounded at both ends by inverted repeats— identical or very similar sequences of nucleotides in reversed orientation. Inverted repeats are usually about 15 to 25 base pairs long and vary among IS elements so that each type of IS has a specific nucleotide sequence in its inverted repeats. Transposase recognizes the ends of the IS and catalyzes transposition. Each IS element is named by giving it the prefix IS followed by a number. IS elements have been observed in a variety of bacteria and some archaea. 23/10/18 10:18 am 16.5 Transposable Elements Move Genes Within and Between DNA Molecules 389 (figure 16.15). Target sites are specific sequences about five to nine base pairs long. When a mobile genetic element inserts at a target site, the target sequence is duplicated so that short, direct-sequence repeats flank the element’s terminal inverted repeats. In replicative transposition, the original transposon remains at the parental site on the chromosome and a copy is inserted at the target DNA site (figure 16.14b). Simple Transposition Transposable elements are of interest for many reasons. Their movement into a chromosome can alter gene function e ither by causing mutations in the genes into which they move or by providing regulatory sites that change the expression patterns of the adjacent genes. They also contribute to the evolution of an organism’s chromosome, plasmids, and other mobile genetic elements. Of particular note is the role of mobile genetic elements in the spread of antibiotic resistance, as we discuss in section 16.9. Insertion sequence DR IR Transposase gene IR Chromosome or plasmid DNA DR (a) Composite transposon Insertion sequence DR IR Transposase gene Insertion sequence IR Antibioticresistance gene IR Transposase gene IR DR (b) Unit transposon DR IR Transposase gene Resolvase gene IR DR (c) Comprehension Check Figure 16.13 Transposable Elements. All transposable elements contain common 1. How does a transposon differ from an insertion sequence? 2. What is simple (cut-and-paste) transposition? What is replicative transposition? How do the two mechanisms of transposition differ? What happens to the target site during transposition? 3. What effect would you expect the existence of transposable elements to have on the rate of microbial evolution? Give your reasoning. features. These include inverted repeats (IRs) at the ends of the element and a recombinase (e.g., transposase) gene. (a) Insertion sequences (IS) consist only of IRs on either side of the transposase gene. (b) Composite transposons and (c) unit transposons contain additional genes (e.g., antibiotic-resistance genes) in addition to the recombinases that enable them to transpose. In composite transposons, the additional genes are flanked by insertion sequences, which supply the transposase. Unit transposons are not associated with insertion sequences. DRs, direct repeats in host DNA, flank a transposable element. MICRO INQUIRY What features are common to all types of transposable elements? Transposons are more complex in structure than IS elements. Some transposons (composite transposons) consist of a central region containing genes unrelated to transposition (e.g., antibiotic-resistance genes) flanked on both sides by IS elements that are identical or very similar in sequence (figure 16.13b). The flanking IS elements encode the transposase used by the transposon to move. Other transposons (unit transposons) lack IS elements and encode their own transposition enzymes (figure 16.13c). Most transposon names begin with the prefix Tn. Two major transposition methods have been identified: simple transposition and replicative transposition (figure 16.14). Simple transposition is also called cut-andpaste transposition. In this method, transposase catalyzes excision of the transposable element, followed by cleavage of a new target site and ligation of the element into this site wil11886_ch16_375-404.indd 389 TE + + Target DNA + (a) Simple transposition Target DNA + (b) Replicative transposition Figure 16.14 Comparison of Simple and Replicative Transposition. TE, transposable e lement. 23/10/18 10:19 am 390 CHAPTER 16 | Mechanisms of Genetic Variation TE—A few hundred to several thousand base pairs in length 5′ 3′ 3′ 5′ 16.6 Bacterial Conjugation Requires Cell-Cell Contact After reading this section, you should be able to: IR Transposase recognizes the inverted repeats and cleaves at both ends of the transposable element, releasing it from its original site. IR 5′ 3′ 3′ 5′ Transposase TE IR IR Transposase carries the TE to a new site and cleaves the target DNA at staggered sites. 3′ 5′ A T G C T G A T A C Target DNA 3′ 5′ The TE is inserted into the target site. 5′ 3′ A A T A C G T T G C 3′ 5′ IR IR TE DNA gap is filled. 5′ 3′ T A T G C A T A C G A T G C T A C G A T 3′ 5′ IR IR TE Direct repeats Figure 16.15 Simple Transposition. IR, inverted repeat; TE, transposable element. wil11886_ch16_375-404.indd 390 a. Identify the type of plasmids that are important creators of genetic variation b. Describe the features of the F factor that allow it to (1) transfer itself to a new host cell and (2) integrate into a host cell’s chromosome c. Outline the events that occur when an F+ cell encounters an F− cell d. Distinguish F+, Hfr, and F ′ cells from each other e. Explain how Hfr cells arise f. Outline the events that occur when an Hfr cell encounters an F− cell Conjugation, the transfer of DNA by direct cell-to-cell contact, depends on the presence of a conjugative plasmid. Recall from chapter 3 that plasmids are small, double-stranded DNA molecules that can exist independently of host chromosomes. They have their own replication origins, replicate autonomously, and are stably inherited. Some plasmids are episomes, plasmids that can exist either with or without being integrated into host chromosomes. Perhaps the best-studied conjugative plasmid is the F factor. It plays a major role in conjugation in E. coli, and it was the first conjugative plasmid to be described (figure 16.16). The F factor is about 100,000 bases long and bears genes responsible for cell attachment and plasmid transfer between specific E. coli cells. Most of the information required for plasmid transfer is located in the tra operon, which contains at least 28 genes. Many of these direct the formation of sex pili that attach the F+ cell (the donor cell containing an F plasmid) to an F− cell (figure 16.17). Other gene products aid DNA transfer. In addition, the F factor has several IS elements that assist plasmid integration into the host cell’s chromosome. Thus the F factor is an episome that can exist outside the bacterial chromosome or be integrated into it (figure 16.18). The initial evidence for bacterial conjugation came from an elegant experiment performed in 1946 by Joshua Lederberg and Edward Tatum. They mixed two auxotrophic strains, incubated the culture for several hours in nutrient medium, and then plated it on minimal medium. To reduce the chance that their results were due to a reversion or suppressor mutation, they used double and triple auxotrophs on the assumption that two or three simultaneous reversion or suppressor mutations would be extremely rare. When recombinant prototrophic colonies appeared on the minimal medium after incubation, they concluded that the two auxotrophs were able to associate and undergo recombination. Lederberg and Tatum did not directly prove that physical contact of the cells was necessary for gene transfer. This evidence was provided several years later by Bernard Davis, who constructed a U-tube consisting of two pieces of curved glass tubing fused at the base to form a U shape with a glass filter between the halves. The filter allowed passage of media but not bacteria. The U-tube was filled with a growth medium and each side inoculated with a different auxotrophic strain of E. coli. During incubation, the medium was pumped back and forth through the filter to ensure exchange. 23/10/18 10:19 am 16.6 Bacterial Conjugation Requires Cell-Cell Contact 391 F factor oriT Encodes pilin protein Encode proteins that are components of a type IV secretion system X tra I trb tra H D tra T tra tra S G tra N trb tra E F trb A tra Q trb trbB trbJ F tra H tra trb tra I W tra U trb C C tra or traiT M tra traJ traY traA traL E tra K tra B tra P trb trbD tra G V tra R Region of F factor with genes needed for conjugation Encodes relaxase Encodes coupling protein Figure 16.16 The F plasmid. Transfer (tra) genes are shown in red, and some of their functions are indicated. The plasmid also contains three insertion sequences and a transposon. The site for initiation of rolling-circle replication and gene transfer during conjugation is oriT. auxotrophy (i.e., chromosomal genes usually were not transferred). However, F− strains frequently became F+. These results are now understood and readily explained in the following way. An F+ strain contains an extrachromosomal F factor O ▼ When the bacteria were later plated on minimal medium, Davis discovered that if the two auxotrophic strains were separated from each other by the filter, gene transfer did not take place. Therefore direct contact was required for the recombination that Lederberg and Tatum had observed. F+ × F− Mating: Plasmid Only Transfer 1 In 1952 William Hayes demonstrated that the gene transfer observed by Lederberg and Tatum was unidirectional. That is, there were definite donor (F+, or fertile) and recipient (F−, or nonfertile) strains, and gene transfer was nonreciprocal. He also found that in F+ × F− mating, the progeny were only rarely changed with regard to 2 IS A F factor B IS Bacterial chromosome ▼ O 1 2 A IS 2 O ▼ A B 1 IS B Integrated F factor Sex pilus Figure 16.17 Bacterial Conjugation. An electron micrograph of two E. coli cells in an early stage of conjugation. The F+ cell to the left is covered with fimbriae, and a sex pilus connects the two cells. ©Dennis Kunkel Microscopy, Inc./Phototake wil11886_ch16_375-404.indd 391 Figure 16.18 F Plasmid Integration. The reversible integration of an F plasmid or factor into a host bacterial chromosome. The process begins with association between plasmid and bacterial insertion sequences. Note that the ISs (insertion sequences) are used for recombination, not transposition. The O arrowhead (white) indicates the site at which oriented transfer of chromosome to the recipient cell begins. A, B, 1, and 2 represent genetic markers. MICRO INQUIRY What does the term episome mean? 23/10/18 10:19 am 392 CHAPTER 16 | Mechanisms of Genetic Variation F factor Bacterial chromosome Coupling factor F+ cell Origin of transfer T4SS Sex pilus makes contact with F- recipient cell. Recipient cell (F–) Relaxosome Frecipient Relaxosome makes a cut at the origin of transfer and begins to separate one DNA strand. The intact strand is replicated by the rolling-circle mechanism. Sex pilus contracts, bringing cells together. Relaxase Accessory proteins of the relaxosome are released. The DNA/relaxase complex is recognized by the coupling factor and transferred to the T4SS. Coupling factor Secretion system The T4SS pumps the DNA/relaxase complex into the recipient cell. T4SS is constructed and joins two cells. Figure 16.19 F Factor–Mediated Conjugation. The F factor encodes proteins for building the sex pilus and the type IV secretion (T4SS) system that anchors the donor to the F− recipient and transfers DNA. One protein, the coupling factor, escorts the DNA to the T4SS. During F+ × F− conjugation, only the F factor is transferred because the plasmid is extrachromosomal. The recipient cell becomes F+. carrying the genes for sex pilus formation and plasmid transfer. The sex pilus is used to establish contact between the F+ and F− cells (figure 16.19). Once contact is made, the pilus r etracts, bringing the cells into close physical contact. The F+ cell prepares for DNA transfer by assembling a type IV secretion system, using many of the same proteins in the sex pilus membrane-spanning structure (figure 16.20). The term sex pilus refers to the extracellular structure, while the membrane-bound components are termed the type IV secretion system (T4SS). Although the two structures share polypeptide components and have complementary roles in conjugation, pilus formation and DNA transfer are independent processes. Conjugation: Transfer of the F Plasmid As the F plasmid is being transferred, it is also copied u sing a process called rolling-circle replication. One strand of the circular DNA is nicked, and the free 3′-hydroxyl end is extended wil11886_ch16_375-404.indd 392 As the DNA enters, the F factor DNA is replicated to become double stranded. F+ cell F+ cell by replication enzymes (figure 16.21). The 3′ end is lengthened while the growing point rolls around the circular template and the 5′ end of the strand is displaced to form an ever-lengthening tail, much like the peel of an apple is d isplaced by a knife as it is 23/10/18 10:19 am 16.6 Bacterial Conjugation Requires Cell-Cell Contact 393 Figure 16.20 The Conjugation Machinery Encoded by F Factor Is a Type IV Secretion System. The F factor–encoded type IV secretion system (T4SS) is composed of numerous Tra proteins, including TraA, which forms the sex pilus, and TraD, the coupling factor. The core complex is used for both pilus biogenesis and DNA transfer. LPS, lipopolysaccharide; OM, outer membrane; P, periplasm; PG, peptidoglycan; PM, plasma membrane. Pilus TraA (pilin) Core complex LPS PG OM P PM TraD (coupling factor) Nick 3′ H O 5′ P pared. We are concerned here with rolling-circle replication of a plasmid. However, rolling-circle replication is also observed during the replication of some v iral genomes (e.g., phage lambda). Rolling-Circle Replication During conjugation, rolling-circle replication is initiated by the relaxosome, a complex of proteins encoded by the F factor (figure 16.16). The relaxosome nicks one strand of the F factor at a site called oriT (for origin of transfer). The major component of the relaxosome is a protein called TraI. It has relaxase activity and remains attached to the 5′ end of the nicked strand. As the F factor is replicated, TraI guides the displaced strand through the T4SS to the recipient cell. It is believed that both ATP hydrolysis and the proton motive force provide the energy for DNA translocation. During plasmid transfer, the entering strand is copied to produce double-stranded DNA. When this is complete, the F− recipient cell becomes F+. Growing point 3′ ATPase 5′ Displaced strand 3′ 5′ Hfr Conjugation Transfers Chromosomal DNA By definition, an F+ cell has the F factor separate from the chro mosome, so in an F+ × F− mating, chromosomal DNA is not transferred. However, within this population, a few cells have the F plasmid integrated (i.e., recombined) into their chromosomes. This explains why not long after the discovery of F+ × F− mating, a second type of F factor–mediated conjugation was discovered. In this type of conjugation, the donor transfers chromosomal genes with great efficiency but does not change the recipient bacteria Displaced strand is almost 1 unit length. 5′ Displaced strand is > 1 unit length. Figure 16.21 Rolling-Circle Replication. A single-stranded tail, often 3′ Complementary strand synthesis 3′ 5′ wil11886_ch16_375-404.indd 393 composed of more than one genome copy, is generated and can be converted to the double-stranded form by synthesis of a complementary strand. The “free end” of the rolling-circle strand is bound to the relaxosome. OH 3′ is the 3′-hydroxyl and P 5′ is the 5′-phosphate created when the DNA strand is nicked. 23/10/18 10:19 am 394 CHAPTER 16 | Mechanisms of Genetic Variation F + cell Hfr cell into F+ cells. Because of the high f requency of recombinants produced by this mating, it is referred to as Hfr conjugation and the Bacterial chromosome donor is called an Hfr strain. pro+ Integration of pro+ Hfr strains contain the F factor integrated into their chromolac+ F factor into lac+ some, rather than free in the cytoplasm (figure 16.22). When intechromosome grated, the F plasmid’s tra operon is still functional; the plasmid can Origin of Origin of direct the synthesis of pili, carry out rolling-circle replication, and transfer transfer transfer genetic material to an F− recipient cell. However, rather than F factor transferring just itself, the F factor also directs the transfer of the host chromosome. DNA transfer begins when the integrated F factor Figure 16.22 Insertion of an F Factor into the Donor Cell’s Chromosome is nicked at oriT. As it is replicated, the F factor begins to move into Creates an Hfr Cell. the recipient (figure 16.23). Initially only part of the F factor is transferred, followed by the donor’s chromoNew strand synthesized some. If the cells remain connected, the entire by rolling-circle replication – Hfr cell F cell chromosome with the rest of the integrated F factor will be transferred; this takes about 100 minutes to accomplish. However, the connecpro– pro– tion between the cells usually breaks before this + + Transfer of Hfr pro pro process is finished. Thus a complete F factor is chromosome + lac – lac – lac lac+ rarely transferred, and the recipient remains F−. lac+ When an Hfr strain participates in pro+ conjugation, bacterial genes are transferred to the recipient in either a clockwise or a counterclockwise direction around a circular Origin of chromosome, depending on the orientation of Conjugation for transfer longer times (toward lac+) the integrated F factor. After the replicated donor chromosome enters the recipient cell, Hfr cell F – recipient it may be degraded or incorporated into the Hfr cell F – recipient Conjugation for F− genome by recombination. Transfer short time of Chromosomal DNA – F΄ Conjugation Because the F factor is an episome, it can leave the bacterial chromosome and resume status as an autonomous plasmid. Sometimes during excision an error occurs and a p ortion of the chromosome is excised, becoming part of the F plasmid. Because this erroneously excised plasmid is larger and genotypically distinct from the original F factor, it is called an F′ plasmid (figure 16.24a). A cell containing an F′ plasmid retains all of its genes, although some of them are on the plasmid. It mates only with an F− recipient, and F′ × F− conjugation is similar to an F+ × F− mating. Once again, the plasmid is transferred as it is copied by rolling-circle replication. Bacterial genes on the chromosome are not transferred (figure 16.24b), but bacterial genes on the F′ plasmid are transferred. These genes need not be incorporated into the recipient chromosome to be expressed. The recipient becomes F′ and is partially diploid because the same bacterial genes present on the F′ plasmid are also found on the recipient’s wil11886_ch16_375-404.indd 394 pro+ lac+ pro– pro+ lac – lac+ pro lac – pro+ lac+ lac+ Recombination between donor DNA and recipient DNA Recombination between donor DNA and recipient DNA pro+ lac+ pro– lac+ pro+ lac+ pro+ lac+ Hfr X F– conjugation Figure 16.23 Hfr × F− Conjugation. Shown are the two cells after initial contact and elaboration of the T4SS. As illustrated, during Hfr × F− conjugation, some plasmid genes and some chromosomal genes are transferred to the recipient. Note that only a portion of the F factor moves into the recipient. Because the entire plasmid is not transferred, the recipient remains F−. In addition, the incoming DNA must recombine with the recipient’s chromosome if it is to be stably maintained. 23/10/18 10:19 am Hfr F′ 16.7 Bacterial Transformation Is the Uptake of Free DNA from the Environment 395 A A Deintegration including part of bacterial chromosome (a) Cells connected by type IV secretion system _ F′ F A factor), as well as other T4SS proteins. Unlike Gram-negative bacteria, which establish contact by way of the sex pilus, cell-to-cell contact in these Gram-positive bacteria is established by surface substances that enable cells to directly adhere to one another. One of the best-studied Gram-positive systems is that of Enterococcus faecalis. The recipient cells of this bacterium release short peptides that activate transfer genes in donor cells containing the proper plasmid. The conjugation systems of Streptomyces spp. differ from those of other Gram-positive bacteria in that T4SS, TraI homologues, and a specific nick site on the plasmid have not been identified. Comprehension Check a F′ plasmid begins replication and transfer. A 1. What is bacterial conjugation and how was it discovered? 2. For F+, Hfr, and F− strains of E. coli, indicate which acts as a donor during conjugation, which acts as a recipient, and which transfers chromosomal DNA. 3. Describe how F+ × F− and Hfr conjugation processes proceed, and distinguish between the two in terms of mechanism and the final results. 4. Compare and contrast F+ × F− and F′ × F− conjugation. 16.7 Bacterial Transformation Is a the Uptake of Free DNA from the Environment After reading this section, you should be able to: F′ plasmid replicated and transferred F′ A F′ A a (b) Figure 16.24 F′ Conjugation. (a) Due to an error in excision, the A gene of an Hfr cell is incorporated into the F factor. (b) During conjugation, the A gene is transferred to a recipient, which becomes diploid for that gene (i.e., Aa). chromosome. In this way, specific bacterial genes may spread rapidly throughout a bacterial population. Bacterial Conjugation in Gram-Positive Bacteria Although most research on plasmids and conjugation has been done using E. coli and other Gram-negative bacteria, conjugative plasmids are present in numerous Gram-positive bacteria. With one exception (Streptomyces spp.), Gram-positive conjugative systems share similarities with those of Gram-negative bacteria. For instance, they have homologues of TraI (relaxase) and TraD (coupling wil11886_ch16_375-404.indd 395 a. Describe the factors that contribute to a bacterium being naturally transformation competent b. Predict the outcomes of transformation using a DNA fragment versus using a plasmid c. Design an experiment to transform bacteria that are not naturally competent with a plasmid that carries genes encoding ampicillin resistance and the protein that generates green fluorescence Another HGT mechanism is transformation, discovered by Fred Griffith in 1928. Transformation is the uptake of circular or linear DNA from the environment outside the cell and maintenance of the DNA in the recipient cell in a heritable form. Natural transformation is distinct from artificial transformation, a laboratory technique that induces cells to take up DNA. Natural transformation has been observed in some archaea and in members of several bacterial phyla. It occurs in soil and aquatic ecosystems, in vivo during infection, and in biofilm and other microbial communities. Natural transformation is best studied in Streptococcus pneumoniae, Bacillus subtilis, and Neisseria spp. It occurs when bacteria lyse and release their DNA into the surrounding environment. These fragments may be relatively large and contain several genes. If a fragment contacts a c ompetent cell—a cell that is able to take up DNA and be transformed—the DNA is bound to the cell and imported (figure 16.25a). The transformation frequency of very competent cells is around 10−3 for most genera when an excess of DNA is used. That is, about one cell in every thousand will take up and integrate the gene. Competency is a complex phenomenon that is induced in about 10 to 15% of a population, during a certain stage of growth. For 23/10/18 10:19 am 396 CHAPTER 16 | Mechanisms of Genetic Variation instance S. pneumoniae becomes competent during early exponential phase, whereas B. subtilis becomes competent in stationary phase. Competence genes require activation through alternative sigma factors or transcription activator proteins. A few species are constitutively competent (e.g., Neisseria spp. and Thermus spp.). DNA fragments Most bacteria are able to take up DNA from any source (i.e., other bacteria, archaea, and eukaryotes). Some take up DNA only from closely related species. For instance, Haemophilus influenzae only takes up DNA containing a specific 9-base-pair sequence that is repeated more than 1,400 times in the H. influenzae genome. Mechanisms used for global regulation (section 14.5) Natural transformation is a multistep process (figure 16.26). Double-stranded (ds) DNA first binds a transformation pilus, lac+ Receptor lac _ DNA fragment binds to a transformation pilus. Bacterial chromosome lac+ Uptake of DNA lac _ One strand is degraded and a single strand is transported into the cell. OR Integration by homologous recombination Degradation Stable transformation An extracellular endonuclease cuts the DNA into smaller fragments. Uptake system lac _ lac+ The DNA strand aligns itself with a homologous region on the chromosome. Unsuccessful transformation lac+ (a) Transformation with DNA fragments lac _ DNA plasmid The DNA strand is incorporated into the chromosome via homologous recombination. Bacterial chromosome Heteroduplex The heteroduplex DNA is repaired using the new DNA as template to create a lac+ gene. Uptake of plasmid Transformed cell lac+ Stable transformation (b) Transformation with a plasmid Figure 16.25 Bacterial Transformation. Transformation with (a) DNA fragments and (b) plasmids. Transformation with a plasmid often is induced artificially in the laboratory. The transforming DNA is in purple, and integration is at a homologous region of the genome. wil11886_ch16_375-404.indd 396 Figure 16.26 Bacterial Transformation in S. pneumoniae. MICRO INQUIRY According to this model, what would happen if DNA that lacked homology to the S. pneumoniae chromosome were taken into the cell? 23/10/18 10:19 am 16.8 Transduction Is Virus-Mediated DNA Transfer 397 Gram-positive bacteria Gram-negative bacteria PilE pilus dsDNA ComGC pilus PilQ Outer membrane PG ComEA ComE PG EndA ComGB Membrane ComEC Cytosol ComGA ComFA PilG Plasma membrane ComA Cytosol PilF ? ssDNA 3′ 3′ Figure 16.27 DNA Uptake Systems of Gram-Positive and Gram-Negative Bacteria. Competence (Com) proteins are related to type II secretion systems. Pilin (Pil) proteins are related to type IV pili. ComFA, ATPase; EndA, endonuclease; PG, peptidoglycan. which directs the DNA to a membrane complex that includes a DNA receptor, an endonuclease, and a transmembrane pore. The nuclease degrades one strand and the remaining intact strand is translocated to the cytoplasm using energy from ATP hydrolysis. The incoming single-stranded (ss) fragment interacts with proteins including RecA that integrate the DNA into the recipient’s chromosome by homologous recombination. Despite the difference in cell envelope architecture, Grampositive and Gram-negative bacteria use similar proteins as the main components of their DNA uptake machinery. These proteins are related to those used in type II secretion systems and type IV pili (see figure 13.43). (Note that type IV pili are unrelated to type IV secretion systems.) The major differences arise from the need for Gram-negative bacteria to move dsDNA across the outer membrane prior to its translocation as ssDNA across the plasma membrane (figure 16.27). Despite knowing the basic machinery, scientists still have not determined the precise mechanisms by which DNA enters the cell or how each component interacts with the DNA. Bacterial Transformation Microbial geneticists exploit transformation to move DNA (usually recombinant DNA) into cells. Because many species, including E. coli, are not naturally transformation competent, these bacteria must be made artificially competent by certain treatments. Two common techniques are electrical shock and exposure to calcium chloride. Both approaches render the cell membrane temporarily more permeable to DNA, and both are used with E. coli. To increase the transformation frequency with E. coli, strains unable to produce one or more nucleases are used. These strains are especially important when transforming the cells with linear DNA, which is vulnerable to attack by nucleases. It is easier to transform bacteria with plasmid DNA because plasmids replicate within the host and wil11886_ch16_375-404.indd 397 are not as easily degraded (figure 16.25b). binant DNA into host cells (section 17.1) Introducing recom- Comprehension Check 1. What is the relationship between transformation and competence? 2. Describe how transformation occurs in S. pneumoniae. How does the process differ in H. influenzae and B. subtilis? 3. Discuss two ways in which artificial transformation can be used to place functional genes within bacterial cells. 16.8 Transduction Is Virus-Mediated DNA Transfer After reading this section, you should be able to: a. Differentiate generalized transduction from specialized transduction b. Correlate a phage’s life cycle to its capacity to mediate generalized or specialized transduction c. Draw a figure, create a concept map, or construct a table that distinguishes conjugation, transformation, and transduction Transduction is mediated by viruses. It is a frequent mode of HGT in nature. Indeed evidence suggests that the number of genes moved by marine viruses from one host cell to another is huge (perhaps 1024 per year). Furthermore, v iruses in marine environments and hot springs move genes b etween organisms in all three domains of life. Marine viruses: mortality at sea (section 30.2) 23/10/18 10:19 am 398 CHAPTER 16 | Mechanisms of Genetic Variation Recall from chapter 6 that virus particles are structurally simple, often composed of just a nucleic acid genome protected by a protein coat called the capsid. Viruses are unable to multiply autonomously. Instead, they infect and take control of a host cell, forcing it to make many copies of the virus. Viruses that infect bacteria are called bacteriophages, or phages for short. Virulent bacteriophages multiply in their bacterial host immediately after entry. After the progeny phage particles reach a certain number, they cause the host to lyse, so they can be released and infect new host cells (see figure 6.16). Thus this process is called the lytic cycle. Temperate bacteriophages, on the other hand, do not immediately kill their host. Instead, the phage establishes a relationship with their host called lysogeny, and bacteria that have been lysogenized are called lysogens. Many temperate phages establish lysogeny by inserting their genomes into the bacterial chromosome. The inserted viral genome is called a prophage. The host bacterium is unharmed by this, and the phage genome is replicated along with the host cell’s genome. Temperate phages may remain inactive in their hosts for many generations. However, they can be induced to switch to a lytic cycle under certain conditions, including UV irradiation. When this occurs, the prophage is excised from the bacterial genome and the lytic cycle proceeds. There are several types of viral infections (section 6.4) Transduction is the transfer of bacterial or archaeal genes by virus particles. It is important to understand that host genes are packaged in the virus particle because of errors made during the virus’s life cycle. The virion containing these genes then transfers them to a new cell. Two kinds of transduction have been described: generalized and specialized. Generalized Transduction Transfers Any Donor Gene Generalized transduction most often occurs during the lytic cycle of virulent phages but sometimes happens during the lytic cycle of temperate phages. Any part of the bacterial genome can be transferred after being partially degraded as the virus takes control of its host (figure 16.28). During the assembly stage, viral genomes are packaged by the “headful mechanism”; that is, only genomes of a certain size (i.e., number of nucleotides in length) are packaged. During generalized transduction, a fragment of the host genome that happens to be about the same size as the phage genome is mistakenly packaged. Such a phage is called a generalized transducing particle, because once it is released, it may encounter a susceptible host cell and eject the bacterial DNA it carries into that cell. However, because it lacks viral genes this does not initiate a lytic cycle. As in transformation, once the DNA fragment has been released into the recipient cell, it must be incorporated into the recipient cell’s chromosome to preserve the transferred genes. The DNA remains double stranded during transfer, and both strands are integrated into the recipient’s chromosome. About 70 to 90% of the transferred DNA is wil11886_ch16_375-404.indd 398 Phage DNA his+ lys+ Phage infects bacterial cell. Host DNA is hydrolyzed into pieces, and phage DNA and proteins are made. his+ lys+ Phages assemble; occasionally a phage carries a piece of the host cell chromosome. lys+ Transducing phage with host DNA his+ Transducing phage injects its DNA into a new recipient cell. his+ Crossing-over Recipient cell _ _ (his lys ) _ his _ lys The transduced DNA is recombined into the chromosome of the recipient cell. _ Recombinant bacterium his+ lys _ The recombinant bacterium has a genotype (his+lys ) _ _ that is different from recipient bacterial cell (his lys ). Figure 16.28 Generalized Transduction in Bacteria. not integrated but often is able to remain intact temporarily and be expressed. Abortive transductants are bacteria that contain this nonintegrated, transduced DNA and are partial diploids. Specialized Transduction Transfers Specific Donor Genes In specialized transduction, only specific portions of the bacterial genome are carried by transducing particles. Specialized transduction is made possible by an error in the lysogenic life 23/10/18 10:19 am 16.8 Transduction Is Virus-Mediated DNA Transfer 399 cycle of temperate phages that insert their genomes into a specific site in the host chromosome. When a prophage is induced to leave the host chromosome, excision is sometimes carried out improperly. The resulting phage genome contains portions of the bacterial chromosome (about 5 to 10% of the bacterial DNA) next to the integration site, much like the situation with F′ plasmids (figure 16.29). However, the transducing particle is defective because it lacks some viral genes and cannot reproduce without assistance. In spite of this, it will inject the remaining viral genome and any bacterial genes it carries into another bacterium. The bacterial genes may become stably incorporated under the proper circumstances. The best-studied example of specialized transduction is carried out by the E. coli phage lambda. The lambda genome inserts into the host chromosome at specific locations known as attachment or att sites (figure 16.30). The phage att sites and bacterial att sites are similar and can complex with each other. The att site for lambda is between the gal and bio genes on the E. coli chromosome; consequently when lambda excises incorrectly to generate a specialized transducing particle, these b acterial genes are most often present. The product of cell lysis (lysate) resulting from the induction of a population of lysogenized E. coli cells contains normal phage and a few d efective transducing particles. These particles are called lambda dgal if they carry the galactose utilization genes or lambda dbio if they carry the bio from the other side of the att site (figure 16.30). Bacteriophage lambda: a temperate bacteriophage (section 26.2) Specialized Transduction Comprehension Check 1. Describe generalized transduction and how it occurs. What is an abortive transductant? 2. What is specialized transduction and how does it come about? 3. How might one tell whether horizontal gene transfer was mediated by generalized or specialized transduction? 4. Why doesn’t a cell lyse after successful transduction with a temperate phage? 5. Describe how conjugation, transformation, and transduction are similar. How are they different? Prophage Lysogenized cell with prophage Induction Rare deintegration that includes some bacterial genes Replication of defective virus DNA with incorporated host genes Assembly and release of transducing phage particles Infection of recipient cell Crossover to integrate bacterial genes Figure 16.29 Specialized Transduction by a Temperate Bacteriophage. Recombination can produce two types of transductants. Integration as prophage MICRO INQUIRY Compare the number of transducing particles that arise during generalized (figure 16.29) and specialized transduction. Why is there such a big difference? wil11886_ch16_375-404.indd 399 Bacterial chromosome containing only donor DNA Bacterial chromosome containing both virus and donor DNA 23/10/18 10:19 am 400 CHAPTER 16 | Mechanisms of Genetic Variation 3 2 gal + gal + 1 Figure 16.30 The Mechanism of Transduction for Phage 1 λ att sites bio+ Integration to form prophage 2 3 bio+ Error in excision Normal excision 3 2 1 Lambda and E. coli. Integrated lambda phage lies between the gal and bio genes. When it excises normally (top left), the new phage is complete and contains no bacterial genes. Rarely, excision occurs asymmetrically (top right), and either the gal or bio genes are picked up and some phage genes are lost (only aberrant excision involving the gal genes is shown). The result is a defective lambda phage that carries bacterial genes and can transfer them to a new recipient. MICRO INQUIRY Why can’t the gal and bio genes be transduced by the same transducing particle? 1 gal + 2 gal + 3 Within 3 years after the widespread use of penicillin began in the 1940s, penicillin-resistant bacteria were found in clinical specimens. To understand the origin of drug resistance, it is important to re1 call that in nature, antibiotic-producing microbes 2 3 1 λ must also protect themselves from the antibiotics λdgal they secrete. In other words, microbes capable of 2 gal + synthesizing antibiotics must also have mechanisms to resist their effects—otherwise they would 3 gal + “commit suicide” by producing the antibiotic. In antibiotic-producing microorganisms, the genes that encode resistance proteins are often referred to as immunity genes. Immunity genes are usually coordinately regulated with genes that code for an1 tibiotic biosynthetic enzymes. It is believed that 2 + many genes encoding antibiotic resistance in bacgal teria were “captured” from antibiotic-producing bacteria and moved by HGT to nonproducers, givgal – ing rise to a large pool of resistance-encoding genes outside the producing microorganisms. It is therefore not surprising that in nonproducing bacteria, genes for drug resistance may be present on bacterial chromosomes, plasmids, transposons, gal + and other mobile genetic elements. Because they Stable transductant are often found on mobile genetic elements, they can easily exchange between bacteria. In addition to acquiring resistance genes by HGT, some nonproducers can become resistant due to spontaneous chromosomal mutations. Usually such mutations result in a change in the drug target; therefore the antibiotic cannot bind and inhibit growth. There are several mechanisms of drug resistance (section 9.8) Frequently a bacterial pathogen is drug resistant because it has a plasmid bearing one or more resistance genes; such plasAfter reading this section, you should be able to: mids are called R plasmids (resistance plasmids; figure 16.31). a. Describe an R plasmid and its associated genetic elements Plasmid-borne resistance genes often code for enzymes that b. Distinguish integrative conjugative elements, transposons, and destroy or modify drugs. Plasmid-associated genes have been conjugative plasmids implicated in resistance to aminoglycosides, chloramphenicol, c. Describe how genetic elements mobilize portions of chromosomes penicillins, cephalosporins, erythromycin, tetracyclines, 16.9 Evolution in Action: The Development of Antibiotic Resistance in Bacteria wil11886_ch16_375-404.indd 400 23/10/18 10:19 am 16.9 Evolution in Action: The Development of Antibiotic Resistance in Bacteria 401 RTF IS1 IS1 Cm Km Amp Sm, Su Tn4 Tn3 38 base pair inverted repeat Target site flanking repeat tnpA Transposase (MW 120 K) tnpR Resolvase (MW 21 K) 38 base pair inverted repeat Target site bla flanking repeat β-lactamase (MW 20 K) Figure 16.31 An R Plasmid. Plasmid R1 is an R plasmid that contains the replicative transposon Tn3. Tn3 contains the gene for β-lactamase (bla), an enzyme that confers resistance to ampicillin (Amp). The arrows below the Tn3 genes indicate the direction of transcription. Note that Tn3 is inserted into another transposable element, Tn4. Tn4 carries genes that provide resistance to streptomycin (Sm) and sulfonamide (Su). The R1 plasmid also carries resistance genes for kanamycin (Km) and chloramphenicol (Cm). The RTF region of R1 codes for proteins needed for plasmid replication and transfer. MICRO INQUIRY As a replicative transposon, what would happen if Tn3 “hopped” from this R1 plasmid into a different plasmid? sulfonamides, and others (see table 9.1). Once a bacterial cell possesses an R plasmid, the plasmid (or its genes) may be transferred to other cells quite rapidly through HGT. Because a single plasmid may carry genes for resistance to several drugs, a pathogen population can become resistant to several antibiotics simultaneously, even though a patient may be treated with only one drug. Antibiotic-resistance genes can be located on genetic elements other than plasmids. Many transposons contain genes for antibiotic resistance and can move rapidly between plasmids and through a bacterial population. As seen in figure 16.31, some resistance genes reside on a transposon. Tn5, a transposon active in many Gram-negative bacteria, is of particular note because it is a replicative transposon. Thus it not only moves but also leaves a copy of itself in its original location. Antibiotic-resistance genes also reside in and move with genetic elements termed integrative conjugative elements (ICEs) wil11886_ch16_375-404.indd 401 an