Week 7 Lecture 11 PDF
Document Details
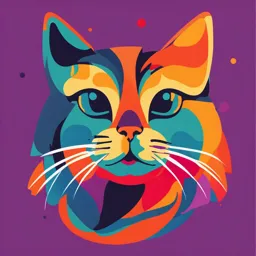
Uploaded by BenevolentRapture
Tags
Summary
This document discusses the role of autophagy in aging and its potential consequences. It also examines the effects of periodic fasting, and encompasses a range of biological processes. Its contents appear to be a set of lecture notes.
Full Transcript
Week 7 Lecture 11 Failure of macroautophagy in aging. Possible causes and consequences of the failure of macroautophagy in old organisms are depicted in this schematic model (brown boxes). (a) The accumulation of autophagic vacuoles with age could result from the inability of lipofuscin- loaded lys...
Week 7 Lecture 11 Failure of macroautophagy in aging. Possible causes and consequences of the failure of macroautophagy in old organisms are depicted in this schematic model (brown boxes). (a) The accumulation of autophagic vacuoles with age could result from the inability of lipofuscin- loaded lysosomes to fuse with autophagic vacuoles and degrade the sequestered content. (b) In addition, the formation of autophagosomes in old cells might be reduced because of the inability of macroautophagy enhancers (such as glucagon) to induce full activation of this pathway. The stimulatory effect of glucagon is compromised in old cells because of maintained negative signaling through the insulin receptor (IR) even under basal conditions (i.e. in the absence of insulin). Maintained insulin signaling would activate mTOR, a known repressor of macroautophagy. Failure of macroautophagy in aging. Possible causes and consequences of the failure of macroautophagy in old organisms are depicted in this schematic model (brown boxes). (c) Inadequate turnover of organelles, such as mitochondria, in aging cells could increase levels of free radicals that generate protein damage and (d) could also potentiate the inhibitory signaling through the insulin receptor. (e) An age-dependent decline in macroautophagy can also result in energetic compromise of the aging cells Autophagy participates in multiple processes that are essential for longevity. Some of the major known mechanisms that regulate autophagy in multiple organisms and their influence on the process. Researchers discovered that feeding mice a calorie-restricted diet that mimics fasting for just eight days a month promoted regeneration in multiple systems and extended longevity. When mice were fed a Fasting Mimicking Diet (FMD) starting at middle age for only four days, twice per month, stem cell numbers increased considerably and various cell types -- such as bone, muscle, liver, brain, and immune cells -- were regenerated. Many of these regenerative effects had not been demonstrated in previous chronic or short-term dietary restriction studies. The animals also experienced better health and a prolonged lifespan, with benefits including reduced inflammatory diseases and cancer, improved learning and memory, and retarded bone loss, without a decrease in muscle mass. Low lysosomal pH (4-5) is maintained by the V-ATPase (vacuolar type ATPase) that uses energy from ATP hydrolysis to constitutively pump H + into the lumen. The low pH is supported by a counter-ion flux involving either the efflux of cations or influx of anions. The low pH of the lysosome maintains the activity of various acid hydrolases (red text) in the luminal fluid that break down the macromolecules that reach lysosomes by various endocytic uptake pathways and autophagy. The building blocks generated from this hydrolysis are exported out of lysosomes via numerous transporters of the SLC (solute carrier) family. Known solutes for the SLC transporters are provided in parentheses next to transporter names. The best described example is the amino acid transporter SLC38A9 that senses luminal arginine to activate mTOR on the lysosomal surface via complexes collectively termed the "amino acid sensing machinery." Cholesterol efflux is also well- described and is exported from lysosomes by NPC1/NPC2 proteins as well as SCARB2. In general, solute efflux is critical for nutrient acquisition but also to prevent an osmotic pressure that could increase lysosomal hydrostatic pressure and membrane tension. Unlike most amino acid transporters in the exchange of Na+ with amino acid symporters, proton-coupled amino acid transporters function as H+ with amino acid symporters. They are located within the luminal surface of the small intestine and within lysosomes, so their action functions in absorption in the intestine and in the efflux pathway after intralysosomal digestion. Unlike typical mammalian amino acid transporters which function in exchanging Na+/amino acid symporters, these- transporters function in exchanging H+/amino acid symporters. In order to grow and divide, cells must increase production of proteins, lipids, and nucleotides while also suppressing catabolic pathways such as autophagy. mTORC1 plays a central role in regulating all of these processes and therefore controls the balance between anabolism and catabolism in response to environmental conditions. mTORC1 promotes protein synthesis largely through the phosphorylation of two key effectors, p70S6 Kinase 1 (S6K1) and eIF4E Binding Protein (4EBP). mTORC1 directly phosphorylates S6K1. S6K1 phosphorylates and activates several substrates that promote mRNA translation initiation, including eIF4B, a positive regulator of the 50cap binding eIF4F complex. mTOR Complex 1 (mTORC1) is composed of the mTOR protein complex, regulatory-associated protein of mTOR (commonly known as raptor), mammalian lethal with SEC13 protein 8 (MLST8), PRAS40 and DEPTOR.This complex embodies the classic functions of mTOR, namely as a nutrient/energy/redox sensor and controller of protein synthesis.The activity of this complex is regulated by rapamycin, insulin, growth factors, phosphatidic acid, certain amino acids (e.g., L-leucine and Arginine), mechanical stimuli, and oxidative stress. The mTORC1 substrate 4EBP is unrelated to S6K1 and inhibits translation by binding and sequestering eIF4E to prevent assembly of the eIF4F complex. mTORC1 phosphorylates 4EBP at multiple sites to trigger its dissociation from eIF4E, allowing 50cap-dependent mRNA translation to occur. Although it has long been appreciated that mTORC1 signaling regulates mRNA translation, whether and how it affects specific classes of mRNA transcripts has been debated. Global ribosome footprinting analyses, however, revealed that, while acute mTOR inhibition moderately suppresses general mRNA translation. Amino Acid Sensing and the Lysosome (C) (1) Ragulator releases GTP from RagC; (2) SLC38A9 is activated by arginine in the lysosome; (3) SLC38A9 converts RagA from the GDP- to the GTP-bound state, leading to the activation of the Rags; (4) Ragulator and SLC38A9 recruit the mTORC1 to the lysosomal surface; and (5) mTORC1 is activated. Kitada et al, Front. Cell Dev. Biol., 2020; https://doi.org/10.3389/fcell.2020.00715 mTORC1 positively regulates several steps in ribosome biogenesis, including ribosomal RNA transcription, the synthesis of ribosomal proteins and other components required for ribosome assembly. mTORC1 can thus coordinate stimuli which promote ribosome production with the various steps involved in this process. mTORC1 regulates mRNA translation on ribosomes and mTOR‐responsive mRNA elements. mTORC1 signaling to S6K and to 4E‐BP1 or 4E‐BP2 to liberate eIF4E can result in increased biogenesis of ribosomes and translation of target mRNAs. mTOR, mechanistic/mammalian target of rapamycin mTORC1 positively regulates several steps in ribosome biogenesis, including ribosomal RNA transcription, the synthesis of ribosomal proteins and other components required for ribosome assembly. mTORC1 can thus coordinate stimuli which promote ribosome production with the various steps involved in this process. mTORC1 regulates mRNA translation on ribosomes and mTOR‐responsive mRNA elements. mTORC1 signaling to S6K and to 4E‐BP1 or 4E‐BP2 to liberate eIF4E can result in increased biogenesis of ribosomes and translation of target mRNAs. mTOR, mechanistic/mammalian target of rapamycin The Fatty Acid-Carnitine Shuttle Dan Foster and Denis McGarry UT Southwestern Schooneman M G et al. Diabetes 2013 Fatty acids derived from chylomicrons, VLDL, or Non- Esterified fatty acids (NEFAs) are transported into cells through CD36 or FATP4. In the cytoplasm fatty acid is converted to a fatty acyl-CoA, followed by conversion to fatty acyl-carnitine through CPT1. CPT2 converts fatty acyl-carnitine to fatty acyl-CoA, which can undergo beta-oxidation to generate acetyl-CoA. All Reactions Occur Between α and β Carbons β-carbon α-carbon Fatty Acid Metabolism Fatty Acid Oxidation - Beta Oxidation Enzymes in Mitochondria and Peroxisomes Generates More ATP Per Carbon Than Sugars Proceeds 2 Carbons at a Time α β Fatty Acid Metabolism Preparation for Oxidation Before Oxidation, Fatty Acids Must be Activated and Transported to the Mitochondrion. Activation Begins in the Cytoplasm Long Chain Fatty Acyl-CoA Ligase Fatty Acid Fatty Acyl-CoA Four Steps in Fatty Acid Oxidation Fatty Acid Metabolism Beta Oxidation - Reaction 1 Oxidation Creates Trans Intermediate Unrelated to Trans fat Three forms of Enzyme Medium Form Problem in SIDS Used to Generate ATP in Oxidative Phosphorylation β α Fatty Acid Metabolism Beta Oxidation Reaction 2 Similar to Fumarase Reaction of Citric Acid Cycle Preparation for Next Oxidation Step β β α α Fatty Acid Metabolism Beta Oxidation Reaction 3 Similar to Malate Dehydrogenase Reaction of Citric Acid Cycle Used to Generate ATP in Oxidative Phosphorylation β β α α Fatty Acid Metabolism Beta Oxidation Reaction 4 Thiolase Enzyme Catalyzes the Reverse Reaction When the R-Group is a Hydrogen Oxidized in Citric Acid Cycle β α Shortened by 2 Carbons Fatty Acid Metabolism Fatty Acid Oxidation - Summary In Summary - Each Round of Oxidation Creates One FADH2, One NADH, one Acetyl-CoA, and a Fatty Acid Shortened by Two Carbons Each Acetyl-CoA Released in Matrix of Mitochondrion Where it is Readily Oxidized in the Citric Acid Cycle.