DNA Tumor Viruses - Rotheneder PDF
Document Details
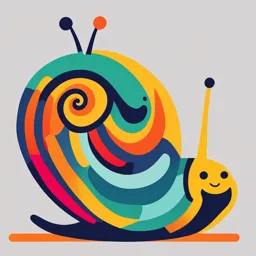
Uploaded by PoisedTimpani1881
Rotheneder
Tags
Summary
This document presents an overview of cancer causes, incidence, and global trends, focusing in particular on Austria. It highlights the significant role of lifestyle factors, environmental exposures, and healthcare infrastructure in cancer development. The document also discusses risk factors and cancer prognosis, as well as advancements in cancer treatment and prevention.
Full Transcript
DNA tumor viruses – Rotheneder Cancer is a significant cause of death in Austria, representing one of the leading mortality factors alongside cardiovascular diseases. As the Austrian population ages, the incidence of cancer-related deaths continues to increase, reflecting broader global trends. Au...
DNA tumor viruses – Rotheneder Cancer is a significant cause of death in Austria, representing one of the leading mortality factors alongside cardiovascular diseases. As the Austrian population ages, the incidence of cancer-related deaths continues to increase, reflecting broader global trends. Austria experiences a high incidence of cancer, with certain cancers such as colorectal, breast, prostate, and lung cancer being the most prevalent. The incidence rates vary by age and gender, with prostate cancer being more common in men and breast cancer in women. Cancer incidence shows geographic variability within Austria, influenced by factors such as lifestyle, access to healthcare, and environmental exposures. However, mortality rates do not always correspond directly to incidence, suggesting differences in healthcare infrastructure and early detection efforts across regions. Globally, cancer incidence varies widely, but mortality rates are more uniform. In regions such as sub-Saharan Africa, infection-related cancers are more prevalent, while in developed nations, cancers associated with lifestyle factors, such as lung and colorectal cancer, dominate. Lung cancer is a leading cause of cancer death worldwide, except in sub-Saharan Africa. Cancer risk increases significantly with age. The probability of developing cancer is approximately 43.5% for men and 38.5% for women from birth to death. This reflects the cumulative effect of mutations and environmental exposures over time. Cancer is often described as a disease of aging. Around the age of 20, there is a notable shift from childhood cancers like leukemia to adult cancers such as those affecting the digestive tract, prostate, lung, and breast. This is attributed to the accumulation of mutations over time. Cancer prognosis varies significantly by type. Breast and prostate cancers have relatively high survival rates due to advancements in early detection and treatment, while lung and pancreatic cancers remain more lethal. Advancements in cancer treatment, such as targeted therapies and immunotherapy, have significantly improved survival rates for certain cancers. Early detection through screening programs has also contributed to better outcomes, particularly for breast and prostate cancer. While incidence rates for some cancers continue to rise, mortality rates have stabilized or declined for certain types, reflecting the impact of improved treatments and early detection. However, disparities remain in less accessible regions. Approximately 25% of cancers are attributed to genetic factors, while 75% are induced by environmental and lifestyle factors such as smoking, diet, and infections. This highlights the significant role of modifiable risk factors in cancer prevention. Cancer risk varies among different tissues, largely explained by the number of stem cell divisions. Tissues with higher rates of cell division have a higher likelihood of accumulating mutations, increasing cancer risk. Exogenous factors such as smoking, alcohol consumption, diet, and infections play a substantial role in cancer development. This reinforces the importance of public health interventions aimed at modifying these risk factors. Lifestyle factors, including tobacco use, poor diet, lack of physical activity, and alcohol consumption, are major contributors to cancer risk. Smoking alone is linked to several cancers, including lung, mouth, esophageal, and bladder cancer. Key recognized cancer risk factors include: - Tobacco (leading cause) - Diet (high consumption of processed meats and low intake of fruits/vegetables) - Infections (e.g., HPV, hepatitis B and C, and EBV) - Reproductive and hormonal factors - Occupational exposures - Alcohol consumption - Pollution - Medical procedures (e.g., radiation exposure) Lung, colorectal, breast, and prostate cancers account for the majority of cancer deaths worldwide. Lung cancer remains the most lethal, with high mortality rates despite advances in treatment. Tobacco use is the most well-known risk factor for cancer, linked to approximately 22% of cancer deaths globally. It is directly responsible for cancers of the lung, mouth, esophagus, and bladder. A critical distinction in cancer epidemiology is understanding correlation versus causation. While many factors correlate with cancer risk, establishing causality requires comprehensive epidemiological and experimental evidence. For instance, while processed meat consumption correlates with colorectal cancer, controlled studies are necessary to confirm direct causation. Tobacco remains one of the most significant risk factors for cancer. Smoking is directly linked to multiple cancers, including lung, mouth, esophagus, stomach, bladder, kidney, and leukemia. The document highlights that smoking dramatically increases relative risk, with lung cancer being the most strongly associated. Tobacco not only introduces carcinogens that cause direct DNA damage but also weakens the immune system’s ability to fight cancer cells. Efforts to reduce smoking rates have led to declines in lung cancer incidence in many regions, yet tobacco remains a primary preventable cause of cancer. Consumption of processed and red meats is linked to an increased risk of colorectal cancer, as supported by numerous epidemiological studies. The document references international comparisons indicating a correlation between meat consumption and cancer incidence, reinforcing the importance of dietary habits. High-temperature cooking methods, such as grilling, can produce carcinogenic compounds like heterocyclic amines (HCAs) and polycyclic aromatic hydrocarbons (PAHs), further elevating cancer risk. Cancer prevention strategies emphasize lifestyle modification. The document lists several preventive measures, including: - Avoid Smoking – Reduces risk for lung, throat, and mouth cancers. - Healthy Diet – Minimizing red/processed meat and increasing intake of fruits and vegetables. - Physical Activity – Regular exercise lowers the risk of colorectal, breast, and endometrial cancers. - Regular Medical Check-Ups – Early detection through screening increases survival rates. - Limit Alcohol Consumption – Reduces risk of liver, throat, and breast cancers. - Avoiding Excess Sun Exposure – Prevents skin cancers like melanoma. - Vaccinations – HPV vaccines lower the risk of cervical and other cancers, while hepatitis B vaccines reduce liver cancer risk. Several infections are directly linked to cancer development. Human papillomavirus (HPV) causes cervical and head/neck cancers, while hepatitis B and C viruses are associated with hepatocellular carcinoma. Epstein-Barr virus (EBV) is linked to Burkitt’s lymphoma and nasopharyngeal carcinoma. Additionally, Helicobacter pylori infection increases the risk of gastric cancer. The document emphasizes the role of vaccinations and antimicrobial treatments in reducing infection-driven cancers. Breast cancer is the most common cancer among women in Europe, accounting for 26.5% of new cases and 17.5% of cancer deaths. Incidence increases with age, peaking after 50. Younger women diagnosed with breast cancer tend to have more aggressive forms. Early detection through mammography significantly improves survival rates, contributing to declining mortality despite rising incidence. The document emphasizes that cancer is fundamentally a disease of aging. The probability of developing cancer rises sharply after 60, with men facing a 43.5% lifetime risk and women 38.5%. This age-related risk reflects the cumulative accumulation of mutations and the declining efficiency of DNA repair mechanisms over time. Cancer’s prevalence in older populations is attributed to the time required for multiple mutations to accumulate in a single cell. This aligns with the multi-step model of carcinogenesis, where successive genetic alterations drive tumor formation. Aging also reduces immune surveillance, allowing abnormal cells to evade detection and proliferate. Frequency of DNA mutations: an estimated 10,000 to 100,000 lesions occur per cell daily. However, most of these are repaired, and only a small fraction lead to permanent genetic changes. Despite this, the large number of dividing cells and cell divisions over a lifetime means that mutations inevitably accumulate, increasing cancer risk. While the probability of a single mutation hitting a cancer-critical gene per cell division is just 0.008%, the large number of cells undergoing division (4 billion proliferating cells) translates to 320,000 cells acquiring mutations during each round of replication. This cumulative effect underscores the high overall risk of cancer despite seemingly low individual probabilities. Several biological mechanisms reduce the likelihood of cancer despite frequent mutations: - Apoptosis – Cells with oncogene activation often undergo programmed cell death. - Immune Surveillance – The immune system targets and destroys abnormal cells. - Order of Mutations – Mutations must occur in a specific sequence to drive cancer progression. - Repair Mechanisms – DNA repair pathways (e.g., mismatch repair) correct many lesions before they cause harm. Mutations often interact, amplifying or suppressing each other’s effects. For example, an oncogene mutation may drive cell division but simultaneously activate apoptosis unless paired with a mutation in tumor suppressor genes like p53. This interdependence complicates cancer development but also offers therapeutic targets. Diploid cells contain the normal complement of chromosomes, while aneuploid cells exhibit abnormal numbers. Aneuploidy, a hallmark of cancer, leads to genetic instability and promotes tumor evolution by allowing rapid accumulation of mutations. This genomic imbalance facilitates cancer progression and therapy resistance. The Goldilocks hypothesis suggests that genomic instability in cancer must be “just right”—sufficient to drive evolution and adaptation, but not so severe that it triggers cell death. Moderate instability accelerates tumor growth, while excessive instability can be lethal to cancer cells. Tumorigenesis is a multi-step process involving numerous barriers: - Apoptosis – Prevents the survival of mutated cells. - Senescence – Limits the lifespan of cells with oncogenic potential. - DNA Damage Response – Detects and repairs DNA lesions. - Immune System – Suppresses early tumor growth through surveillance. The hallmarks of cancer, originally described by Hanahan and Weinberg, have expanded to include: - Sustaining proliferative signaling. - Evading growth suppressors. - Resisting cell death. - Enabling replicative immortality. - Inducing angiogenesis. - Activating invasion and metastasis. - Deregulating cellular energetics. - Avoiding immune destruction. Cancer evolves through clonal selection, where cells with advantageous mutations outcompete their neighbors. This Darwinian process leads to increasingly aggressive and treatment-resistant tumors over time. Tumor progression is marked by sequential genetic changes, resulting in increased malignancy. Clonal expansions drive the accumulation of mutations, eventually leading to metastasis and treatment resistance. Modern sequencing technologies allow for the identification of “genomic scars” in cancer cells—mutational patterns reflecting past exposures and DNA repair deficiencies. These scars can guide personalized treatment by revealing vulnerabilities unique to each tumor. Two primary gene classes drive cancer: - Proto-Oncogenes – Promote cell division (e.g., MYC, RAS). - Tumor Suppressor Genes – Inhibit cell division (e.g., p53, RB1). Cancer arises when proto-oncogenes are activated or tumor suppressors are inactivated, disrupting the balance of cellular proliferation. Proto-oncogenes are normal genes that regulate cell growth and division. They play essential roles in cell signaling, proliferation, and differentiation. When mutated or dysregulated, proto-oncogenes can become oncogenes, leading to uncontrolled cell growth and contributing to cancer development. Examples include: - Growth Factors and Receptors – e.g., PDGF (platelet-derived growth factor). - Signal Transduction Proteins – e.g., Ras family genes. - Transcription Factors – e.g., MYC. - Cell Cycle Regulators – e.g., cyclins and CDKs. Proto-oncogenes can transform into oncogenes through various mechanisms: - Point Mutations – A single base change can hyperactivate the gene (e.g., Ras mutation). - Gene Amplification – Increased copies of a proto-oncogene lead to overexpression (e.g., MYCN in neuroblastoma). - Chromosomal Translocations – Rearrangement of chromosomes places a proto- oncogene under the control of an active promoter. - Insertional Mutagenesis – Viral insertion near a proto-oncogene can enhance its expression. A hallmark of chronic myelogenous leukemia (CML) is the translocation between chromosomes 9 and 22, creating the Philadelphia chromosome. This results in the formation of the BCR-ABL fusion protein. BCR-ABL is a constitutively active tyrosine kinase that drives unregulated cell division by bypassing normal growth signals. - Clinical Relevance – BCR-ABL is targeted by imatinib (Gleevec), a tyrosine kinase inhibitor, which significantly improves CML outcomes. While oncogenes promote cell division when overactive, tumor suppressor genes act as brakes to inhibit proliferation and prevent cancer. The balance between oncogene activation and tumor suppressor inactivation determines cancer development. - Example: p53 functions as a tumor suppressor by inducing cell cycle arrest and apoptosis. When mutated, p53 loses its suppressive role, contributing to cancer progression. Tumor suppressor genes regulate the cell cycle, repair DNA, and ensure genomic stability. Loss of function in TSGs leads to unregulated growth and cancer. Common TSGs include: - RB (Retinoblastoma protein) – Regulates the G1/S checkpoint. - p53 – Oversees DNA damage responses and induces apoptosis. - BRCA1/BRCA2 – Involved in DNA repair, particularly in breast and ovarian cancer. Tumor suppressors are categorized based on their roles: - Gatekeepers – Directly control cell proliferation by regulating the cell cycle. (e.g., pRB). - Caretakers – Maintain genomic stability by repairing DNA damage. (e.g., BRCA1, p53). - Landscapers – Regulate the extracellular environment to prevent tumor formation. (e.g., PTEN). Tumor suppressors are often inactivated in cancer through two primary mechanisms: - Mutations – Inactivating mutations can occur in both alleles (loss of heterozygosity), eliminating the gene’s function. - Epigenetic Modifications – Promoter methylation silences tumor suppressor genes without altering DNA sequences. Mutations leading to loss of function are common in TSGs. For example: - p53 mutations often occur in the DNA-binding region, preventing proper transcriptional activation of downstream genes involved in apoptosis and cell cycle arrest. - RB gene mutations lead to retinoblastoma and other cancers. Promoter methylation silences tumor suppressor genes by preventing transcription. This epigenetic mechanism frequently affects genes like p16INK4a and BRCA1. In colon cancer, hypermethylation of the MLH1 gene is linked to mismatch repair deficiency, contributing to microsatellite instability. SIRT6, a member of the sirtuin family, is involved in: - Inhibiting Aerobic Glycolysis – Suppresses the Warburg effect, which is common in cancer metabolism. - Enhancing DNA Repair – Promotes genomic stability by regulating repair pathways. - Regulating MYC and HIF-1α – Inhibits pathways essential for tumor growth. Loss of SIRT6 promotes tumor progression, while its overexpression reduces tumorigenesis. A specific SIRT6 variant (N308K/A313S), enriched in centenarians, enhances genome stability and increases interaction with Lamin A, a nuclear envelope protein linked to longevity. This variant improves DNA repair, reducing the risk of age-related cancers. The Adenomatous Polyposis Coli (APC) gene is a tumor suppressor crucial for colorectal cancer prevention. APC mutations lead to uncontrolled cell growth in the colon by disrupting WNT signaling. - Pathogenesis – Loss of APC is often the initiating event in colorectal cancer. Colon carcinoma progresses through stages: - Hyperplasia – Increased cell proliferation. - Adenoma (Polyp) – Benign glandular growth. - Carcinoma in Situ – Localized invasive cancer. - Metastasis – Cancer spreads to other organs. APC interacts with various cellular proteins, including β-catenin. Mutations result in the accumulation of β-catenin, driving the transcription of oncogenic genes. APC also regulates cell adhesion and migration. FAP is an inherited disorder caused by germline APC mutations, leading to hundreds of polyps in the colon by early adulthood. If untreated, nearly all FAP patients develop colorectal cancer. Prophylactic colectomy is often recommended. APC is a key regulator of the WNT pathway. In the absence of WNT signals: - APC forms a complex with axin, GSK3β, and β-catenin to degrade β-catenin. - WNT Activation – Disrupts the APC complex, allowing β-catenin to enter the nucleus and activate genes driving proliferation. Mutations in APC prevent β-catenin degradation, leading to excessive cell growth. β-catenin plays a dual role: - Cell Adhesion – Links cadherins to the cytoskeleton. - Transcription Factor – When stabilized, β-catenin promotes proliferation. In colonic crypts, APC tightly regulates β-catenin. Dysregulation causes crypt expansion and polyp formation, progressing to carcinoma. Cell cycle checkpoints are surveillance mechanisms that ensure the accurate replication and division of cells by monitoring DNA integrity. If damage or replication errors are detected, checkpoints halt the cell cycle to allow for repair or trigger apoptosis if the damage is irreparable. These checkpoints prevent the propagation of mutations that could lead to cancer. The cell cycle is regulated by four major checkpoints: 1. G1/S Checkpoint (Restriction Point): - Ensures that the cell is ready for DNA replication. - Checks for DNA damage and sufficient cell size. - Key regulators: p53 (induces cell cycle arrest via p21) and Rb (Retinoblastoma protein). 2. S-Phase Checkpoint: - Monitors DNA replication for errors. - Prevents replication stress and ensures all DNA is accurately copied. - Regulators: ATR (Ataxia Telangiectasia and Rad3-related) and Chk1. 2. G2/M Checkpoint: - Ensures DNA has been fully replicated without damage before mitosis. - Halts mitosis if double-strand breaks (DSBs) or incomplete replication are detected. - Key proteins: ATM (Ataxia Telangiectasia Mutated), Chk2, and p53. 4. Spindle Assembly Checkpoint (SAC): - Occurs during metaphase to ensure proper attachment of chromosomes to the spindle. - Prevents aneuploidy by delaying anaphase if chromosomes are misaligned. - Key regulators: Bub1, Mad2, and Aurora kinases. DNA damage can result from endogenous and exogenous sources: - Endogenous Causes: Replication errors, reactive oxygen species (ROS), and spontaneous mutations. - Exogenous Causes: UV radiation, ionizing radiation, chemicals (mutagens), and viruses. Unrepaired DNA damage can lead to genomic instability, cell death, or malignant transformation. Accumulation of DNA lesions, especially DSBs, is a primary driver of cancer development. Certain inherited syndromes predispose individuals to cancer by impairing DNA repair pathways: - Xeroderma Pigmentosum (XP): Defect in nucleotide excision repair (NER). - Li-Fraumeni Syndrome: Mutations in p53. - Ataxia Telangiectasia (A-T): Caused by mutations in ATM. - BRCA1/2 Mutations: Lead to breast and ovarian cancer due to homologous recombination repair defects. XP is a rare autosomal recessive disorder characterized by extreme sensitivity to UV radiation, leading to skin cancers at a young age. XP results from mutations in genes involved in NER, preventing the repair of UV-induced pyrimidine dimers. Without repair, these lesions accumulate, causing mutations that drive carcinogenesis. Double-strand breaks (DSBs) are among the most lethal forms of DNA damage. The DSB model posits that DSBs activate a robust signaling cascade that halts the cell cycle and initiates repair. This response involves: - Sensing the Break: The MRN complex (MRE11, RAD50, NBS1) detects DSBs. - Signaling the Damage: ATM is recruited and activated at the site of damage. - Effector Activation: ATM phosphorylates downstream proteins, including Chk2 and p53, to mediate repair or apoptosis. Two Main Mechanisms of Double-Strand Break Repair 1. Homologous Recombination (HR): - Error-free repair using a sister chromatid as a template. - Occurs during S and G2 phases when sister chromatids are available. - Key proteins: BRCA1, RAD51, and PALB2. 2. Non-Homologous End Joining (NHEJ): - Error-prone process that directly ligates broken DNA ends. - Active throughout the cell cycle but predominant in G1 phase. - Key proteins: Ku70/Ku80 complex, DNA-PKcs, and XRCC4. A-T is an autosomal recessive disorder caused by mutations in the ATM gene. It leads to progressive neurodegeneration, immune deficiencies, and increased cancer risk. Patients exhibit sensitivity to ionizing radiation due to impaired DSB repair. ATM is a serine/threonine kinase that plays a central role in the DNA damage response (DDR). It is activated by DSBs and orchestrates cell cycle arrest, DNA repair, and apoptosis. ATM Structure and Post-Translational Modifications - Structure: ATM contains FAT, PI3-kinase-like (PIKK), and kinase domains. - Modifications: ATM undergoes autophosphorylation at Ser1981, which activates its kinase function. Additional modifications include acetylation and ubiquitination that fine-tune its activity. Upon DSB formation, ATM is recruited to damage sites by the MRN complex. This recruitment is essential for ATM activation, which leads to phosphorylation of H2AX (forming γH2AX), marking the site for repair protein accumulation. Activated ATM phosphorylates multiple downstream targets, including: - H2AX – Facilitates recruitment of repair proteins. - Chk2 – Mediates cell cycle arrest. - p53 – Induces apoptosis or senescence. - BRCA1/2 – Promotes homologous recombination repair. The MRN complex is essential for detecting DSBs and recruiting ATM: - MRE11: Nuclease activity that processes broken DNA ends. - RAD50: Structural protein that stabilizes DNA. - NBS1: Facilitates ATM recruitment and signal transduction. Players in DNA Damage Checkpoint and Repair - ATM/ATR – Master regulators of DSB and replication stress responses. - Chk1/Chk2 – Effector kinases that enforce cell cycle arrest. - p53 – Tumor suppressor governing apoptosis and repair. - BRCA1/2 – Facilitates HR repair. - Ku70/80 – NHEJ factors that bind broken DNA ends. Chk2 is a checkpoint kinase activated by ATM in response to DSBs. It phosphorylates CDC25 phosphatases, halting cell cycle progression. Chk2 also promotes apoptosis by activating p53 and E2F1. Chk2 activation involves: - Phosphorylation at Threonine-68 (T68) by ATM. - Dimerization and Autophosphorylation – This fully activates Chk2, allowing it to phosphorylate targets involved in cell cycle control and apoptosis. By regulating the DNA damage response, Chk2 plays a pivotal role in preventing genomic instability and cancer development. DNA damage checkpoint and repair involve a complex network of proteins that detect, signal, and repair damage to maintain genomic stability. Key players include: - ATM (Ataxia Telangiectasia Mutated): Responds to double-strand breaks (DSBs). - ATR (Ataxia Telangiectasia and Rad3-Related): Activated by replication stress and single-stranded DNA (ssDNA). - CHK1/CHK2 (Checkpoint Kinases): Enforce cell cycle arrest and DNA repair through phosphorylation of key targets. - p53: Mediates apoptosis and cell cycle arrest in response to DNA damage. - BRCA1/BRCA2: Facilitate homologous recombination (HR) repair. - MRN Complex (MRE11, RAD50, NBS1): Detects DSBs and recruits ATM. - H2AX: Phosphorylated (γH2AX) at damage sites to recruit repair proteins. - CDC25 Phosphatases: Targets of CHK1/CHK2 to delay cell cycle progression. - Ku70/80 and DNA-PKcs: Involved in non-homologous end joining (NHEJ). ATR is a serine/threonine kinase essential for responding to replication stress and single-stranded DNA. It prevents replication fork collapse and ensures complete DNA replication before mitosis. ATR phosphorylates CHK1, triggering a signaling cascade that leads to cell cycle arrest, DNA repair, or apoptosis. - Key Function: ATR protects against genomic instability by stabilizing replication forks and coordinating DNA repair during S-phase. Seckel syndrome is a rare autosomal recessive disorder caused by ATR mutations. It is characterized by: - Microcephaly - Growth retardation - Facial abnormalities (“bird-headed dwarfism”) - Developmental delays Patients with Seckel syndrome experience chromosomal instability and an increased risk of cancer, reflecting ATR’s role in DNA damage repair. Replication stress arises from stalled replication forks, leading to genomic instability. ATR is the central regulator of the Replication Stress Response (RSR). - Mechanism: ATR senses ssDNA coated with RPA (Replication Protein A) and is activated through recruitment by TOPBP1 (Topoisomerase II Binding Protein 1). - Outcome: ATR phosphorylates CHK1 to pause the cell cycle, allowing replication to resume or DNA damage to be repaired. Cancer cells often experience high replication stress, making ATR a therapeutic target. Inhibiting ATR in cancer therapy forces cells into mitotic catastrophe, selectively killing tumor cells. CHK1 is a kinase activated by ATR in response to replication stress. Its main functions include: - Phosphorylation of CDC25A/C: Inhibits CDC25, preventing CDK activation and blocking cell cycle progression at the S and G2 phases. - Replication Fork Stabilization: CHK1 prevents replication fork collapse by regulating proteins involved in fork stabilization. - DNA Repair Coordination: Facilitates homologous recombination and nucleotide excision repair. CHK1 and CHK2 both phosphorylate CDC25 to enforce cell cycle arrest, but they differ in their activation and specific roles: - CHK1: Activated by ATR and primarily responds to replication stress during S- phase. - CHK2: Activated by ATM in response to DSBs during G1/G2. Despite functional overlap, CHK1 is essential for replication fork stability, while CHK2 is crucial for apoptosis and genomic integrity following DSBs. CDC25 phosphatases remove inhibitory phosphates from CDKs, allowing cell cycle progression. CHK1/CHK2 phosphorylate and inhibit CDC25, preventing CDK1 activation and halting the cell cycle at the G2/M checkpoint. - Outcome: DNA repair is prioritized, preventing mitotic entry with damaged DNA. - Cancer Implications: Defects in this pathway allow cells with damaged DNA to proliferate, promoting tumorigenesis. BRCA1 and BRCA2 are tumor suppressor genes critical for DNA repair through homologous recombination. Germline mutations in BRCA1/2 significantly increase the risk of breast and ovarian cancer. - Lifetime Risk: BRCA1 mutations confer up to a 70% risk of breast cancer by age 80. BRCA2 mutations carry a 45-65% risk. - Role in Cancer: Loss of BRCA function leads to genomic instability and accumulation of mutations. Breast cancer is the most common cancer among women in Europe, accounting for: - 350,000 new cases annually. - 26.5% of all female cancers. - 17.5% of female cancer deaths. Mortality is declining due to early detection and improved treatments, but disparities exist across regions. Breast Cancer (Some Demographics) - Incidence Peaks: After age 50, coinciding with menopause. - Early Menstruation and Late Menopause: Increase risk by prolonging exposure to estrogen. - Reproductive Factors: Late pregnancies or nulliparity elevate risk. - Ethnicity: Higher incidence in Western Europe, lower in Eastern Europe and Asia. Inheritance, Family History, and Lifestyle Determine the Risk - Family History: Women with first-degree relatives with breast cancer have a twofold higher risk. - BRCA1/2 Inheritance: Autosomal dominant pattern. - Lifestyle Factors: Obesity, alcohol consumption, and hormone replacement therapy (HRT) also contribute to breast cancer risk. BRCA = Breast Cancer BRCA1/2 mutations are synonymous with hereditary breast and ovarian cancer. Testing and prophylactic measures (e.g., mastectomy) are vital for carriers. Structure of BRCA1 and BRCA2 - BRCA1: Contains RING domains, BRCT domains, and interacts with RAD51 and PALB2. - BRCA2: Facilitates RAD51 recruitment to DNA damage sites. BRCA1 plays diverse roles in: - DNA Repair: Essential for HR repair of DSBs. - Checkpoint Regulation: Enforces G2/M arrest through CHK1 activation. - Transcriptional Regulation: Interacts with p53 to control gene expression post- DNA damage. BRCA1 forms complexes with BARD1, PALB2, and RAD51 to promote DNA repair. It also interacts with H2AX and 53BP1, regulating repair pathway choice. BRCA1 functions as an E3 ubiquitin ligase, tagging proteins for degradation to facilitate DNA repair. Ubiquitylation of histones by BRCA1 is crucial for chromatin remodeling at damage sites. BRCA2’s primary role is to recruit and stabilize RAD51 at DNA breaks to initiate homologous recombination. Upon DSB formation: - BRCA2 binds ssDNA at the break. - RAD51 is loaded onto the DNA, promoting strand invasion and repair. - Homology Search and DNA Synthesis occur using the sister chromatid as a template. Loss of BRCA2 results in faulty repair, promoting chromosomal rearrangements and tumorigenesis. Inactivation of BRCA1/2 genes results in impaired DNA repair, particularly in the homologous recombination (HR) pathway, leading to genomic instability and an increased risk of breast, ovarian, and other cancers. Cells deficient in BRCA1/2 accumulate double-strand breaks (DSBs) that are repaired by error-prone pathways like non-homologous end joining (NHEJ), increasing mutation rates and chromosomal aberrations. Cancer Risk: - BRCA1: Associated with a 70% lifetime risk of breast cancer and 40-50% risk of ovarian cancer. - BRCA2: Conveys a 45-65% risk for breast cancer and 10-20% risk for ovarian cancer. - Prostate and Pancreatic Cancer: Elevated in BRCA2 mutation carriers. The document shows a four-generation pedigree illustrating the inheritance of a BRCA1 mutation. The pattern highlights autosomal dominant transmission, where each child of an affected parent has a 50% chance of inheriting the mutation. Women in the family exhibit a high incidence of breast and ovarian cancer, with some developing cancer at an early age. Retinoblastoma is a rare pediatric cancer of the retina, driven by mutations in the RB1 gene. The RB (Retinoblastoma) protein is a critical tumor suppressor that regulates the cell cycle by controlling the G1/S checkpoint. Loss of RB function leads to unregulated cell division and tumorigenesis. The Knudson “Two-Hit Hypothesis” explains retinoblastoma development: - First Hit: Germline or somatic mutation in one allele of the RB1 gene. - Second Hit: A somatic mutation or loss of the remaining functional allele. - Result: Loss of both RB1 alleles leads to tumor formation. Statistically, hereditary retinoblastoma appears earlier and often bilaterally (both eyes), while non-hereditary cases typically present later and unilaterally. In normal individuals, both copies of the RB1 gene are functional, regulating the cell cycle and preventing excessive proliferation. The RB protein (pRB) binds to E2F transcription factors, keeping them inactive and blocking entry into the S-phase until the cell is ready to divide. Hereditary retinoblastoma occurs when children inherit a defective RB1 allele. A single additional somatic mutation (second hit) is sufficient to inactivate the remaining healthy copy, leading to tumor formation. Characteristics: - Bilateral tumors (both eyes). - Early onset (before age 5). - Increased risk of secondary cancers (osteosarcoma, melanoma). Non-hereditary retinoblastoma arises from two independent somatic mutations in both RB1 alleles within the same retinal cell. - Characteristics: - Unilateral tumors (one eye). - Later onset. Six Ways of Losing the Remaining Good Copy of RB - Nondisjunction (Chromosomal Loss): Loss of the chromosome carrying the wild- type RB1 allele. - Mitotic Recombination: Results in loss of heterozygosity (LOH). - Gene Conversion: During DNA repair, the mutant allele is copied, replacing the normal allele. - Deletion: Large chromosomal deletions affecting the RB1 locus. - Point Mutations: Spontaneous mutations that inactivate the wild-type allele. - Promoter Methylation: Epigenetic silencing of the RB1 gene without altering the DNA sequence. Loss of RB function in retinal cells leads to unchecked proliferation and tumor formation. In retinoblastoma, biallelic RB1 inactivation is a hallmark. Without functional RB1, E2F is constitutively active, promoting continuous cell cycle progression and tumor growth. Retinoblastoma develops through: - Hyperplasia of Retinal Cells. - Formation of Retinal Tumors. - Local Invasion and Metastasis (if untreated). Children with hereditary retinoblastoma are at risk for additional cancers, necessitating early screening and intervention. Laser photocoagulation is a targeted treatment for small, localized retinoblastoma tumors. The laser destroys cancer cells by coagulating blood vessels that supply the tumor. This method is often used in conjunction with chemotherapy or cryotherapy for more advanced cases. RB1 mutations are not limited to retinoblastoma. Loss of RB function is implicated in: - Osteosarcoma. - Small-cell lung cancer. - Bladder cancer. - Breast cancer. In these tumors, RB1 inactivation leads to genomic instability, accelerating tumor progression. The pRB protein belongs to the pocket protein family, which includes p107 and p130. These proteins regulate cell cycle progression by binding to and inhibiting E2F transcription factors. - pRB: Controls the G1/S transition. - p107 and p130: Regulate cell cycle exit and quiescence. pRB forms complexes with various cellular proteins to exert its tumor suppressor function: - Cyclin/CDK Complexes: Phosphorylate and inactivate pRB, releasing E2F. - HDAC (Histone Deacetylase): pRB recruits HDAC to repress transcription. - E2F Family: Interacts directly with E2F to regulate genes required for S-phase entry. pRB binds E2F transcription factors, which regulate the expression of genes essential for DNA synthesis and cell cycle progression. In its active (hypophosphorylated) state, pRB sequesters E2F, preventing uncontrolled proliferation. The E2F family controls the expression of genes involved in cell cycle progression, DNA repair, and apoptosis. - E2F1-3: Activators that drive cell cycle progression. - E2F4-8: Repressors that inhibit proliferation. pRB directly inhibits E2F1-3, halting the G1/S transition. Cell Cycle Control by Pocket Proteins - G0/Early G1: pRB binds to E2F, preventing S-phase entry. - Late G1/S-Phase: Cyclin D/CDK4/6 phosphorylates pRB, releasing E2F and allowing DNA replication. In the more detailed model: - Cyclin D/CDK4/6 initiates pRB phosphorylation. - Cyclin E/CDK2 completes pRB inactivation, fully releasing E2F. - p107 and p130 reinforce cell cycle control by acting at multiple checkpoints, ensuring precise regulation of cell division. The transition from late G1 to the S phase of the cell cycle is a critical checkpoint where the cell commits to DNA replication. This process is tightly regulated by cyclin- dependent kinases (CDKs) and cyclins. - Key Players: Cyclin E/CDK2 phosphorylates pRB (Retinoblastoma protein), releasing E2F transcription factors. Free E2F drives the expression of genes required for DNA synthesis, pushing the cell into the S phase. - Checkpoint Control: The integrity of this checkpoint prevents the replication of damaged DNA, reducing the risk of genomic instability and cancer. E2F transcription factors are central regulators of cell cycle progression, controlling the expression of genes required for DNA replication and mitosis. - Active State: When pRB is phosphorylated, E2F is free to activate target genes. - Repressed State: In early G1, hypophosphorylated pRB binds to E2F, inhibiting transcription and blocking entry into the S phase. - CDK Inhibitors (CKIs): Proteins such as p21 and p27 inhibit CDK activity, maintaining pRB in its repressive state. E2F1 is unique among the E2F family due to its dual role in cell cycle progression and apoptosis. - Cell Cycle Progression: In normal conditions, E2F1 drives the transcription of genes promoting S phase entry. - Apoptosis Induction: Under stress, such as DNA damage, E2F1 activates pro- apoptotic genes (e.g., p53, APAF1, and BAX), leading to cell death. This dual functionality serves as a safeguard to eliminate potentially cancerous cells. E2F1 directly links cell cycle progression to apoptosis by regulating both proliferative and apoptotic pathways. - Mechanism: If DNA damage or oncogene activation occurs, E2F1 activates p53 and other apoptotic regulators. This dual coupling ensures that cells with irreparable damage are eliminated before entering mitosis. E2F1 induces apoptosis by activating transcription of pro-apoptotic genes, including: - p14ARF – Stabilizes p53 by inhibiting MDM2, p53’s negative regulator. - APAF1 – A key player in the apoptosome, initiating the intrinsic apoptosis pathway. - BID/BAX – Promote mitochondrial outer membrane permeabilization (MOMP), triggering cell death. When overexpressed, E2F1 drives the cell cycle forward, leading to uncontrolled S phase entry. This hyperproliferative state can lead to genomic instability, a hallmark of cancer. Paradoxically, excessive E2F1 also triggers apoptosis, highlighting its tumor suppressor role. - Threshold Effect: Moderate E2F1 levels promote proliferation, but excessive levels activate p53 and pro-apoptotic Bcl-2 family members, leading to cell death. In response to DNA damage, E2F1 shifts from a proliferative to an apoptotic or senescence-inducing factor. This transformation prevents the propagation of damaged cells. - Mechanism: DNA damage stabilizes E2F1 by ATM/ATR-mediated phosphorylation, enhancing its interaction with pro-apoptotic genes. Hyperactive oncogenes (e.g., MYC, RAS) drive uncontrolled proliferation, leading to replication stress and DNA damage. This triggers the DNA damage response (DDR), activating p53 and halting the cell cycle or inducing apoptosis. p53 is a tumor suppressor that prevents genomic instability by inducing cell cycle arrest, DNA repair, apoptosis, and senescence. Functions: - Cell Cycle Arrest: Induces p21, inhibiting CDKs and halting the cell cycle at G1/S. - Apoptosis: Activates BAX and NOXA, promoting mitochondrial apoptosis. - Senescence: Drives cells into permanent growth arrest if damage is irreparable. - DNA Repair: Facilitates repair by activating GADD45 and other repair genes. Mutations in TP53 are found in over half of human cancers, making it one of the most frequently mutated genes in oncology. Inactivation of p53 leads to: - Unregulated Growth: Cells bypass cell cycle checkpoints. - Resistance to Apoptosis: Damaged cells evade death, accumulating further mutations. p53 knockout mice exhibit a high incidence of spontaneous tumors, confirming its crucial role in tumor suppression. - Implication: The loss of even a single p53 allele predisposes mice to lymphomas and sarcomas. While mouse models provide valuable insights, they do not perfectly mimic human tumorigenesis. For example: - p53 targets differ between mice and humans. - Cancer latency and tissue specificity vary between species. Structure: p53 consists of: - Transactivation Domain (TAD): Activates target genes. - DNA-Binding Domain: Binds to p53-responsive elements. - Tetramerization Domain: Facilitates p53’s function as a tetramer. - Post-Translational Modifications: - Phosphorylation (by ATM/ATR): Stabilizes p53. - Acetylation: Enhances transcriptional activity. - Ubiquitination (by MDM2): Targets p53 for degradation. Ferroptosis is an iron-dependent form of cell death driven by lipid peroxidation. p53 enhances ferroptosis by repressing SLC7A11, a component of the glutathione anti- oxidant system, increasing lipid ROS and promoting cancer cell death. Most tumor-associated p53 mutations occur in the DNA-binding domain (DBD), impairing its ability to activate target genes. This renders p53 non-functional in suppressing tumor growth. Role of Normal and Mutated p53: - Normal p53: Activates repair, induces apoptosis, and enforces checkpoints. - Mutated p53: Fails to regulate the cell cycle, enabling tumor progression. In some cases, mutated p53 gains oncogenic functions (GOF), promoting cancer development. Consequences of p53 Mutations: - Loss of Function: Cells accumulate DNA damage. - Gain of Function (GOF): Mutant p53 drives metastasis and chemoresistance. Dominant-negative mutations in p53 lead to tetramers composed of mutant and wild- type subunits. This partially or fully impairs the function of the entire complex, contributing to tumorigenesis even with a single mutant allele. Although p53 is primarily known as a tumor suppressor, in certain contexts, mutant forms of p53 can acquire oncogenic functions (GOF - Gain of Function). These mutated p53 proteins not only lose their tumor-suppressive roles but also actively promote tumor progression, metastasis, and chemoresistance. - Mechanism: Mutant p53 binds to and interferes with normal transcription factors, driving uncontrolled proliferation and inhibiting apoptosis. - Clinical Relevance: Tumors harboring p53 mutations often show poorer prognosis and increased resistance to therapy. p53 acts as a cellular stress sensor, responding to various signals that indicate genomic instability or damage. The following stressors activate p53: - DNA Damage: Ionizing radiation, UV light, and chemotherapeutic agents. - Hypoxia: Low oxygen levels activate p53 to prevent cell survival under adverse conditions. - Oncogene Activation: Excessive oncogene activity (e.g., MYC, RAS) triggers p53 to induce cell cycle arrest or apoptosis. - Oxidative Stress: Reactive oxygen species (ROS) accumulation activates p53 to mitigate potential damage. - Nutrient Deprivation: p53 regulates metabolism and autophagy in response to low nutrient levels. p53 functions in a dose-dependent manner, with different cellular outcomes based on its concentration: - Low Levels: Induce cell cycle arrest to facilitate DNA repair. - Moderate Levels: Promote cellular senescence, preventing proliferation. - High Levels: Trigger apoptosis, eliminating cells with severe damage. This gradient of p53 activity ensures a flexible response tailored to the severity of cellular stress. The affinity of p53 for specific gene promoters influences cell fate: - High-Affinity Sites: Promote the transcription of cell cycle arrest genes (e.g., p21). - Low-Affinity Sites: Activate pro-apoptotic genes (e.g., BAX, NOXA). This binding hierarchy means that cells initially attempt to repair damage but switch to apoptosis if stress persists. Mdm2 (Murine Double Minute 2) is the primary negative regulator of p53, functioning as an E3 ubiquitin ligase that targets p53 for degradation. - Feedback Loop: p53 activates Mdm2 transcription, establishing a negative feedback loop to keep p53 levels in check. - Stress Disruption: Under stress, Mdm2 is inhibited (via phosphorylation by ATM/ATR), stabilizing and activating p53. Cellular signals fine-tune p53 activity: - Survival Signals (PI3K/AKT Pathway): Activate Mdm2, promoting p53 degradation and enabling cell survival. - Stress Signals (ATM/ATR): Phosphorylate p53 and Mdm2, inhibiting p53 degradation and promoting repair or apoptosis. - Oncogenic Stress (ARF Pathway): p14ARF binds Mdm2, preventing p53 degradation and amplifying p53’s tumor-suppressive activity. Outcomes of Mdm2-Mediated p53 Ubiquitination: - Low Ubiquitination: Leads to nuclear export of p53, allowing temporary regulation without complete degradation. - Polyubiquitination: Results in proteasomal degradation of p53, suppressing its tumor-suppressive functions. - Monoubiquitination: Alters p53 localization, shifting it to the cytoplasm, where it plays non-genomic roles in apoptosis. WIP1 (Wild-type p53-Induced Phosphatase 1) downregulates the DNA damage response by dephosphorylating key proteins, including p53, ATM, and CHK2. - Function: Terminates the DDR once the damage is repaired, restoring cellular homeostasis. - Cancer Role: Overexpression of WIP1 can lead to premature deactivation of p53, contributing to tumor progression. p53 function is often compromised in cancer through several mechanisms: - Point Mutations: Result in loss or GOF. - Deletion of TP53 Locus: Complete loss of p53 protein. - Mdm2 Overexpression: Leads to enhanced degradation of wild-type p53. - Promoter Methylation: Epigenetic silencing of TP53 transcription. - Post-Translational Modifications: Dysregulated phosphorylation or acetylation impairs p53 stability and function. ZDHHC1 is a palmitoyltransferase that modifies and stabilizes p53 by adding a palmitoyl group, facilitating its localization to the plasma membrane. - Loss of ZDHHC1: Reduces p53 palmitoylation, impairing its tumor-suppressive function. - Implication: Cancer cells lacking ZDHHC1 show diminished p53 activity, enhancing survival and proliferation. Upon DNA damage, p53 transactivates p21 (CDKN1A), a cyclin-dependent kinase inhibitor. Role of p21: - Binds and inhibits CDK2 and CDK4/6, preventing pRB phosphorylation. - Maintains pRB in its active form, halting the cell cycle at the G1/S checkpoint. - Allows DNA repair to proceed without replication of damaged DNA. p53 drives apoptosis through transcriptional activation of pro-apoptotic genes: - BAX: Promotes mitochondrial permeabilization, releasing cytochrome c to initiate apoptosis. - FAS (CD95): Engages the extrinsic apoptosis pathway by activating death receptors. - IGFBP3 (Insulin-Like Growth Factor Binding Protein 3): Inhibits IGF-1 signaling, reducing survival signals and enhancing apoptosis. p53’s role as the “guardian of the genome” lies in its ability to: - Halt the cell cycle in response to DNA damage. - Facilitate repair of damaged DNA. - Induce apoptosis when repair fails. - Suppress oncogenic transformation by neutralizing hyperactive oncogenes. - Modulate metabolism to prevent cancer progression. However, loss or mutation of p53 leads to unregulated growth, resistance to cell death, and increased genomic instability – hallmarks of cancer. MicroRNAs (miRNAs) are small non-coding RNAs that regulate gene expression post- transcriptionally. p53 modulates the expression of various miRNAs, contributing to its tumor suppressor function. In turn, miRNAs can regulate p53 expression and activity by targeting genes involved in its degradation or stabilization. - Key miRNAs regulated by p53: - miR-34a/b/c: Induces apoptosis and cell cycle arrest by targeting CDK4/6 and BCL2. - miR-145: Suppresses oncogenes like MYC and KRAS. - miR-192/miR-215: Reinforce the p53 pathway by promoting cell cycle arrest through p21 (CDKN1A). Reciprocally, miRNAs such as miR-25 and miR-504 inhibit p53 expression, demonstrating a feedback mechanism that fine-tunes p53 activity. Dysregulation of these miRNAs can impair p53’s tumor-suppressive functions, promoting cancer progression. Studies indicate that p53 activation depends on the intensity of oncogenic signals rather than their duration. - Low-Intensity Signals: May induce temporary cell cycle arrest but do not fully activate p53. - High-Intensity Signals: Trigger robust p53 activation, leading to apoptosis or senescence. This highlights how p53’s threshold response protects cells from hyperproliferation driven by strong oncogenic stress, such as MYC overexpression or RAS mutations. Emerging research focuses on re-engineering p53 to enhance its tumor-suppressive abilities. - Strategies include: - Stabilizing wild-type p53 through Mdm2 inhibitors. - Restoring mutant p53 to its functional state using small molecules (PRIMA-1). - Enhancing p53’s pro-apoptotic activity by increasing its affinity for low-affinity target genes like BAX and PUMA. These approaches aim to redirect p53 activity towards apoptosis in cancer cells, bypassing pathways that lead to cell cycle arrest or senescence. Peto’s paradox describes the observation that large animals (e.g., elephants, whales) do not exhibit higher cancer rates despite having more cells. - Resolution: Large animals have evolved enhanced tumor suppressor mechanisms, including multiple copies of the TP53 gene. - Example: Elephants possess up to 20 copies of TP53 (compared to 2 in humans), leading to hyperactive p53 responses that eliminate cells with DNA damage more efficiently. The expansion of TP53 copy numbers in elephants correlates with their increased body size and longevity. This evolutionary adaptation enhances the DNA damage response (DDR), providing greater protection against cancer. - Mechanism: The additional TP53 copies reduce mutation accumulation by promoting apoptosis and repair in precancerous cells. Therapeutic strategies aim to direct p53’s activity toward apoptosis, selectively killing cancer cells while sparing healthy tissue. Approaches include: - Small Molecules (Nutlin-3): Disrupts p53-Mdm2 interactions, stabilizing p53. - Gene Therapy: Introduction of wild-type TP53 into tumors via viral vectors. - Targeting Pro-Apoptotic Pathways: Enhancing BAX, NOXA, and PUMA expression downstream of p53. Despite extensive research, p53’s full range of functions and interactions remains incompletely understood. Challenges include: - Complex regulatory networks involving p53. - Context-dependent roles in apoptosis, senescence, and cell cycle arrest. - Gain-of-function mutations that drive cancer progression. p53 transcriptionally activates numerous genes involved in: - Cell Cycle Arrest: p21, GADD45 - Apoptosis: BAX, PUMA, NOXA - DNA Repair: XPC, DDB2 - Angiogenesis Inhibition: TSP1 p14ARF and p16INK4A are tumor suppressors that act upstream of p53 and pRB (Retinoblastoma protein). - p14ARF: Inhibits Mdm2, stabilizing p53 in response to oncogenic stress. - p16INK4A: Inhibits CDK4/6, preventing pRB phosphorylation and enforcing G1 arrest. Mutations in these pathways frequently occur in human cancers. DNA tumor viruses (e.g., adenoviruses, papillomaviruses) can be used as vectors to deliver therapeutic genes into cancer cells. - Goal: Introduce functional tumor suppressor genes or induce apoptosis in tumor cells. - Vectors: Engineered adenoviruses (Oncorine) deliver wild-type p53 into cancer cells, inducing apoptosis. Classical gene therapy focuses on correcting inherited genetic disorders by inserting functional copies of defective genes. - Example: ADA-SCID (Severe Combined Immunodeficiency) treatment through gene therapy. Cancer gene therapy aims to: - Restore Tumor Suppressor Genes: Introduce functional TP53 into tumors. - Inhibit Oncogenes: Deliver siRNA or shRNA to silence oncogenes like KRAS. - Enhance Immune Response: Use viruses to stimulate anti-tumor immunity. Vectors used in cancer gene therapy include: - Adenoviruses: High efficiency and non-integrative. - Lentiviruses: Integrate into host DNA for long-term expression. - AAV (Adeno-Associated Viruses): Safe and less immunogenic. Tumor formation is not the goal but a byproduct of viral transformation during infection by DNA tumor viruses. - Example: HPV infection can lead to cervical cancer. In productive infection, viruses replicate without integrating into host DNA. However, integration of oncogenic viral genes (E6 and E7 from HPV) can drive malignant transformation. Transformation by HPV E6 and E7 - E6 Protein: Degrades p53 via ubiquitination. - E7 Protein: Inactivates pRB, releasing E2F and promoting unregulated cell division. SV40 large T antigen binds to and inactivates both p53 and pRB, dismantling key tumor suppressor pathways. This results in genomic instability and tumorigenesis. DNA tumor viruses disrupt G1/S checkpoints by targeting p53 and pRB, promoting cell cycle progression even in the presence of DNA damage. This allows for the accumulation of mutations, driving cancer formation. p53, known as the “guardian of the genome,” is often inactivated by cancer cells or viruses to bypass apoptosis and enable uncontrolled proliferation. Two primary mechanisms facilitate the removal of p53: 1. Proteasomal Degradation (Mdm2 Pathway): - Mdm2 is an E3 ubiquitin ligase that binds p53 and ubiquitinates it, marking it for degradation by the proteasome. - In response to DNA damage, p53 induces p14ARF, which inhibits Mdm2, stabilizing p53. However, viruses exploit Mdm2 to suppress p53 activity. 2. Viral Protein Interaction (Sequestration or Direct Degradation): - Adenovirus E1B-55K and E4orf6 proteins form a complex that ubiquitinates p53, leading to its degradation. - HPV E6 protein binds to p53 and, with the help of the cellular E6AP ubiquitin ligase, drives p53 degradation. - SV40 Large T Antigen binds and sequesters p53, preventing it from initiating transcription of pro-apoptotic genes. Adenoviruses are non-enveloped, double-stranded DNA viruses capable of infecting a wide range of human cells. While adenoviruses typically cause mild respiratory infections, their ability to manipulate host cell machinery makes them useful in cancer gene therapy and oncolytic virus development. - Size and Structure: About 90 nm in diameter with a linear, double-stranded DNA genome (~36 kb). - Host Range: Broad, infecting dividing and non-dividing cells. - Oncolytic Potential: Engineered adenoviruses selectively replicate in tumor cells, causing lysis. Life Cycle of Adenoviruses 1. Attachment and Entry: - Adenovirus binds to host cell receptors (e.g., CAR – Coxsackie-Adenovirus Receptor). - Internalization occurs through clathrin-mediated endocytosis. 2. Endosomal Escape: - The viral particle escapes the endosome, releasing viral DNA into the cytoplasm. 3. Entry into the Nucleus: - The viral DNA enters the nucleus through nuclear pores, initiating transcription and replication. 4. Gene Expression and Replication: - Early genes (E1A, E1B) alter the cell cycle, while late genes encode structural proteins. 5. Assembly and Release: - Virus particles assemble in the nucleus and are released by cell lysis. Following endosomal escape, adenovirus DNA associates with nuclear import proteins. Viral DNA enters the nucleus without integrating into the host genome, allowing efficient replication and transcription. Gene Regions of Adenovirus: - Early Genes (E1, E2, E3, E4): Regulate cell cycle progression and inhibit apoptosis. - Late Genes (L1-L5): Encode structural proteins for viral assembly. E1A is the first gene expressed after infection, driving the host cell into the S phase to facilitate viral replication. E1A binds to pRB, releasing E2F transcription factors and promoting cell cycle progression. However, this can induce p53-mediated apoptosis. - E1A triggers apoptosis if the cell senses uncontrolled proliferation, necessitating viral anti-apoptotic measures. E1B-55K and E1B-19K are adenoviral proteins that inhibit apoptosis: - E1B-55K: Binds and inactivates p53. - E1B-19K: A Bcl-2 homolog that blocks mitochondrial apoptosis pathways by inhibiting BAX/BAK. The E1B-55K/E4orf6 complex forms an E3 ubiquitin ligase that targets p53 for degradation, allowing adenoviruses to replicate without triggering apoptosis. This complex also degrades host proteins involved in DNA repair, facilitating viral replication. Adenoviruses suppress apoptosis by: - E1B-55K/E4orf6 complex: Degrades p53. - E1B-19K: Inhibits pro-apoptotic proteins (BAX, BAK). - E4orf3: Alters chromatin to suppress the transcription of p53 target genes. Virus vs. Tumor Cell – Comparison of Armory: - Virus Tools: E1A, E1B, E4orf6, E4orf3. - Tumor Cell Tools: Oncogene activation (e.g., RAS, MYC), p53 inactivation, immune evasion. - Viruses often mimic tumorigenesis pathways, creating competition for host resources. Oncolytic adenoviruses are engineered to selectively replicate in and lyse cancer cells. - Key Strategy: Delete E1B (e.g., ONYX-015), allowing the virus to replicate only in cells with dysfunctional p53. Adenovirus serotype 5 (Ad5) is commonly used for oncolytic and gene therapy vectors due to its well-characterized genome and high infectivity. Genomic Organization of Adenovirus - Early Genes (E1-E4): Hijack the host cell cycle. - Late Genes (L1-L5): Encode capsid proteins and structural components. Examples of Replication-Competent Vectors - ONYX-015: Selective replication in p53-deficient tumor cells. - CG0070: Targets RB pathway-deficient cells by driving E2F-1 expression. In normal cells, ONYX-015 cannot replicate efficiently because it lacks E1B, which is necessary to degrade functional p53. As a result, the virus is eliminated, sparing healthy tissue. E4-ORF3 forms nuclear inclusion bodies that promote histone methylation at p53 target promoters, suppressing apoptosis and DNA repair genes, ensuring efficient viral replication. Tumor cells lacking functional p53 allow ONYX-015 to replicate unchecked, leading to oncolysis. Tumor selectivity arises from the virus’s inability to degrade p53 in normal cells, restricting replication to cancerous tissues. Oncolytic viruses undergo Darwinian selection within tumors, evolving to infect resistant tumor cell clones more effectively, ensuring long-term efficacy. Clinical Studies and Results: - ONYX-015: Showed efficacy in head and neck cancer, with improved survival in combination with chemotherapy. - CG0070: Demonstrated promise in bladder cancer by inducing tumor lysis. Reasons for Higher Success Rates: - Tumor Selectivity: Engineered to target p53/RB-deficient cells. - Combination Therapy: Oncolytic viruses enhance the effectiveness of chemotherapy and radiation. ONYX-015, an oncolytic adenovirus engineered to replicate in p53-deficient tumor cells, showed limited efficacy (14% response rate) when used alone in clinical trials. The reasons for this modest response include: - Immune Clearance: The immune system rapidly eliminates adenoviruses, limiting viral replication and tumor destruction. - Heterogeneous Tumor Profiles: Not all tumors exhibit complete p53 inactivation, restricting ONYX-015 replication. - Replication Barriers: Tumor microenvironments (e.g., hypoxia) impede viral replication and spread. - Inadequate Tumor Lysis: ONYX-015 induces apoptosis, but tumors with robust anti-apoptotic pathways can resist viral oncolysis. - Solution: Combining ONYX-015 with chemotherapy significantly improved outcomes by enhancing tumor cell susceptibility and reducing immune clearance. Oncorine (H101) is the first commercially approved oncolytic adenovirus (China, 2005) and is derived from ONYX-015. - Modification: Deletion of the E1B-55K gene, allowing selective replication in tumor cells with dysfunctional p53. - Clinical Success: Oncorine demonstrated a higher efficacy (30-40%) in combination with chemotherapy for head and neck cancers. KD1 and KD3 are engineered oncolytic adenoviruses designed for greater tumor specificity and enhanced replication. - KD1: E1B-55K deleted, but with additional modifications for improved infectivity. - KD3: Incorporates deletions in E1B and E4 regions to selectively replicate in tumor cells with disrupted cell cycle checkpoints. Infection of Tumor Cells with KD1 or KD3 - Selective Replication: KD1 and KD3 target cells with defective p53 or Rb pathways. - Tumor Lysis: Efficient replication leads to oncolysis and the release of viral progeny, promoting the destruction of surrounding tumor cells. - Immune Response Activation: Tumor cell lysis induces an anti-tumor immune response, enhancing the therapeutic effect. To enhance specificity, oncolytic adenoviruses use tumor-specific promoters that restrict viral gene expression to cancer cells. - Examples of Tumor-Specific Promoters: - hTERT (Telomerase Reverse Transcriptase): Active in most cancer cells but silent in normal cells. - Survivin Promoter: Targets cells with high survivin expression, common in aggressive tumors. - CEA (Carcinoembryonic Antigen) Promoter: Targets colorectal and pancreatic cancers. ICOVIR-7 is an oncolytic adenovirus designed with enhanced tumor selectivity and replication efficiency. - Modifications: Incorporates an E2F-1 promoter to drive E1A expression, ensuring replication in cells with Rb pathway defects. - Clinical Application: Demonstrated efficacy against retinoblastoma and glioblastoma. ICO15K is an optimized adenovirus with increased infectivity and tumor specificity. Enhancements: - RGD motif insertion in the fiber protein, enabling infection through integrins overexpressed in tumor cells. - Tumor-Specific E2F Promoter: Drives replication exclusively in cancer cells. OBP-301 (Telomelysin) is an oncolytic adenovirus driven by the hTERT promoter, selectively targeting cancer cells with high telomerase activity. - Broad Spectrum: Effective in multiple cancer types, including esophageal, lung, and gastric cancers. - Cancer Stem Cell Targeting: OBP-301 targets not only bulk tumor cells but also cancer stem cells (CSCs), which are often resistant to conventional therapies. OBP-702 is an advanced version of OBP-301 that combines telomerase-driven replication with p53 gene therapy. - Mechanism: OBP-702 induces p53 overexpression, restoring tumor suppressor activity in cancer cells. - Dual Action: Simultaneously promotes apoptosis and viral oncolysis, enhancing therapeutic efficacy. CTVs are engineered adenoviruses designed to replicate in tumor cells and simultaneously deliver therapeutic genes. - Example: Ad5-p53 – Combines adenoviral replication with p53 gene therapy, inducing apoptosis and suppressing tumor growth. - Goal: Enhance tumor destruction while reducing off-target effects on normal tissues. Ad-cycE and Rapamycin: - Ad-cycE: An adenovirus driven by the Cyclin E promoter, replicating in cells with deregulated cell cycles. - Rapamycin (mTOR Inhibitor): Combined with Ad-cycE, rapamycin suppresses angiogenesis and tumor proliferation, creating a synergistic anti-cancer effect. Problems with Oncolytic Adenoviruses: - Immune Clearance: Rapid immune detection limits viral replication. - Heterogeneous Tumors: Tumors with intact p53 resist selective replication. - Limited Tumor Penetration: Viral spread is restricted within solid tumors. Overcoming Problems – Alternate Receptors and Serotypes: - Alternate Receptors: Engineering adenoviruses to recognize integrins (RGD modification) or CD46 enhances infection efficiency. - Serotype Switching: Using less immunogenic adenovirus serotypes (e.g., Ad35 or Ad11) reduces immune clearance, improving persistence in the tumor. Strategies to Improve Adenoviral Vector Specificity: - Tumor-Specific Promoters: Limit viral gene expression to cancer cells (e.g., hTERT or survivin promoters). - Conditional Replication: Require specific oncogenic signals (e.g., RAS pathway activation) for viral replication. - Gene Deletions (E1B-55K): Prevent replication in normal cells while allowing tumor replication. Other Strategies to Enhance Oncolytic Adenoviruses: - Combination Therapy: Use with chemotherapy, radiation, or immune checkpoint inhibitors (e.g., anti-PD-1). - Arming Viruses: Incorporate pro-apoptotic or immune-stimulatory genes (e.g., GM-CSF). - Viral Shedding Enhancement: Modify fiber proteins to improve dissemination within tumors. Replication-defective adenoviruses lack essential early genes (e.g., E1 or E3), allowing gene delivery without viral replication. Applications: - Gene Therapy: Delivery of therapeutic genes (e.g., p53, VEGF inhibitors). - Cancer Vaccines: Induce anti-tumor immunity by expressing tumor antigens. - Example: Ad-p53 (Gendicine) – Delivers functional p53 to tumors, promoting apoptosis and restoring cell cycle control. Replication-defective adenoviral vectors are widely used in glioblastoma treatment through suicide gene therapy. These vectors lack essential viral replication genes (e.g., E1A/E1B) but retain the ability to deliver therapeutic genes to tumor cells. - Mechanism: The adenovirus introduces a “suicide gene” into tumor cells, which produces a toxic product when activated by a prodrug. - Target Disease: Glioblastoma, an aggressive brain tumor resistant to conventional therapies, has been a prime candidate for this approach. Suicide gene therapy involves the selective killing of cancer cells by delivering genes that encode for enzymes capable of converting non-toxic prodrugs into lethal compounds. - Gene Delivery: A viral vector delivers a gene (e.g., HSV-tk – Herpes Simplex Virus thymidine kinase). - Prodrug Administration: A non-toxic prodrug (e.g., ganciclovir) is administered systemically. - Enzyme Activation: The enzyme produced by the suicide gene converts the prodrug into a toxic metabolite, inducing apoptosis in tumor cells. Ganciclovir (GCV) is a nucleoside analog used as the prodrug in HSV-tk-based suicide gene therapy. - Mechanism: HSV-tk phosphorylates ganciclovir into a toxic triphosphate form that integrates into DNA, causing chain termination and apoptosis. - Selective Toxicity: Only cells expressing HSV-tk convert ganciclovir into its active form, minimizing systemic toxicity. A critical advantage of ganciclovir therapy is the bystander effect: - How It Works: Cells expressing HSV-tk generate toxic metabolites that diffuse to adjacent non-transduced tumor cells, enhancing therapeutic efficacy beyond directly infected cells. - Importance: This effect addresses the challenge of incomplete vector delivery to all tumor cells, resulting in broader tumor eradication. Cerepro is a replication-defective adenoviral vector carrying the HSV-tk gene, designed for the treatment of glioblastoma. - Administration: Injected directly into the tumor bed following surgical resection. - Mechanism: Upon prodrug (ganciclovir) administration, transduced cells undergo apoptosis, reducing tumor recurrence. A Phase III clinical trial evaluating Cerepro in glioblastoma patients showed: - Extended Survival: Patients receiving Cerepro and ganciclovir had improved survival compared to standard care. - Safety Profile: Well-tolerated with minimal side effects, although the overall benefit was moderate, indicating the need for combination strategies. Many tumors overexpress anti-apoptotic proteins (e.g., Bcl-2), making them resistant to apoptosis. Gene therapies target these pathways to restore apoptotic sensitivity. - Example: Adenoviral vectors delivering p53 or dominant-negative forms of anti- apoptotic proteins (e.g., T34A mutant IκBα) enhance tumor cell death. pAd-T34A is an adenoviral vector expressing a dominant-negative form of IκBα, which inhibits NF-κB, a key regulator of anti-apoptotic signaling. - Mechanism: pAd-T34A suppresses NF-κB, sensitizing tumor cells to apoptosis. - Clinical Effect: In models of disseminated peritoneal cancer, pAd-T34A significantly reduced tumor burden and improved survival. A novel strategy involves viral vectors delivering bacterial toxins (e.g., diphtheria toxin or Pseudomonas exotoxin) directly to tumors. - Mechanism: Toxins disrupt protein synthesis, inducing apoptosis selectively in transduced tumor cells. - Advantages: High potency with minimal systemic toxicity due to tumor-specific promoters controlling toxin expression. Fusion proteins combining viral proteins with tumor-targeting ligands enhance adenoviral vector specificity and transduction efficiency. - Example: Adenoviruses engineered with integrin-binding (RGD) motifs or fibronectin-targeting domains show increased tumor selectivity and uptake. Adenoviral vectors frequently deliver tumor suppressor genes such as p53, RB, or PTEN to restore growth regulation in tumor cells. - Outcome: Re-expression of tumor suppressors halts proliferation, induces apoptosis, and sensitizes cells to chemotherapy. The Ad-p53 trial aimed to evaluate the safety and efficacy of adenoviral p53 gene therapy in patients with advanced cancers. - Goals: - Assess tumor response. - Determine p53 restoration’s impact on apoptosis and cell cycle arrest. - Evaluate combination therapy with chemotherapy or radiation. Gendicine is the first commercially approved gene therapy for cancer, developed in China. It utilizes a replication-defective adenovirus to deliver the wild-type p53 gene to tumor cells. - Indication: Approved for head and neck squamous cell carcinoma (HNSCC). Gendicine: Mode of Action: - Mechanism: Gendicine delivers functional p53 to tumor cells, restoring apoptosis and cell cycle regulation. - Therapeutic Effect: Tumor cells with defective p53 undergo apoptosis, while normal cells remain unaffected. Gendicine: Routes of Administration: - Intratumoral Injection: Direct delivery into solid tumors enhances transduction efficiency. - Intravenous (IV) Delivery: Used for metastatic or disseminated cancers, though less efficient due to immune clearance. Gendicine: Advanced Head and Neck Squamous Cancer and Advanced Hepatic Cancer - Head and Neck Cancer: Clinical trials demonstrated increased response rates when Gendicine was combined with chemotherapy and radiotherapy. - Hepatic Cancer: Ongoing studies show promise in treating hepatocellular carcinoma (HCC) by inducing tumor regression and improving survival. Gendicine is the first gene therapy approved for cancer (China, 2003). It uses a replication-defective adenovirus to deliver the wild-type p53 gene into tumor cells. Production: - Vector: Adenovirus serotype 5 (Ad5). - Gene Insert: Full-length human wild-type p53 cDNA. - Manufacturing: Large-scale production follows Good Manufacturing Practice (GMP) standards to ensure purity, stability, and efficacy. Regulatory Hurdles: - Western Approval Challenges: Despite approval in China, Gendicine faced regulatory obstacles in the US and EU due to concerns over long-term safety, immune responses, and lack of large-scale randomized trials. - Efficacy Data: Critics argued that clinical trial data lacked the rigorous statistical analysis required by Western regulatory agencies. A case report highlighted synergistic effects of Gendicine when combined with conventional anticancer drugs (e.g., cisplatin or 5-fluorouracil). - Findings: Patients with head and neck squamous cell carcinoma (HNSCC) showed enhanced tumor regression and longer progression-free survival compared to chemotherapy alone. Recent studies reaffirmed the efficacy of Gendicine in treating liver, lung, and esophageal cancers. Results: - Tumor Reduction: Significant tumor shrinkage in combination with radiotherapy. - Safety Profile: Minimal systemic toxicity observed. - Immune Response: Enhanced immune infiltration into tumors, suggesting immune-modulatory effects. Talimogene laherparepvec (T-VEC) is the first FDA-approved oncolytic virus (2015) for advanced melanoma. It uses a modified Herpes Simplex Virus Type 1 (HSV-1). Mechanism of Action: - Direct Oncolysis: T-VEC infects and lyses tumor cells. - GM-CSF Expression: The virus expresses granulocyte-macrophage colony- stimulating factor (GM-CSF) to recruit immune cells. - Immune Activation: Cell lysis releases tumor antigens, triggering systemic anti- tumor immunity. T-VEC Improves Anti-PD-1 Immunotherapy: - Combination Therapy: T-VEC combined with anti-PD-1 inhibitors (e.g., nivolumab or pembrolizumab) enhances immune checkpoint blockade. - Rationale: T-VEC increases T-cell infiltration into tumors, overcoming checkpoint resistance. - Clinical Results: - Objective Response Rates (ORR): Nearly doubled in melanoma patients receiving T-VEC + anti-PD-1. - Durable Responses: Improved overall survival and longer-lasting tumor control. CAR T cell therapy involves engineering T cells to express chimeric antigen receptors (CARs) targeting tumor antigens (e.g., CD19, BCMA). - Mechanism: CAR T cells recognize and kill cancer cells through antigen-directed cytotoxicity. - Applications: Approved for B-cell malignancies (e.g., leukemia, lymphoma). - Limitations: Solid Tumor Challenges: Poor infiltration and immunosuppressive tumor microenvironments limit efficacy. - Toxicity: Cytokine release syndrome (CRS) and neurotoxicity are common side effects. Combining oncolytic viruses (OVs) with CAR T cell therapy enhances solid tumor targeting. - Rationale: Oncolytic viruses disrupt the tumor microenvironment, facilitating CAR T cell infiltration. - Example: T-VEC + CAR T Cells: T-VEC infection leads to tumor inflammation, making tumors more susceptible to CAR T cell attack. Bi-specific T-cell engagers (BiTEs) are engineered proteins that bind CD3 (on T cells) and tumor antigens (e.g., CD19, EGFR). - Mechanism: BiTEs recruit T cells to tumors, inducing cytotoxicity. - Advantages: - High Precision: Direct T cell activation against tumor cells. - Approved Therapies: Blinatumomab (anti-CD19 BiTE) is approved for acute lymphoblastic leukemia (ALL). - Expanding Applications: New BiTEs target solid tumors and hematologic malignancies. Autosis is a unique form of cell death driven by autophagy dysfunction, distinct from apoptosis and necrosis. - Myxoma Virus (MYXV): A poxvirus capable of infecting tumor cells while leaving normal cells unharmed. - Combination with CAR-T Cells: - MYXV enhances CAR T cell function, inducing autosis in resistant tumor cells. - Effective against tumors that evade apoptosis. - Clinical Potential: Offers a new pathway for targeting apoptosis-resistant cancers, expanding the therapeutic reach of CAR T and TCR-T cells. Summary of Viral and Immunotherapy Advancements in Cancer Treatment: - Gendicine and Oncolytic Viruses: Viral vectors like Gendicine and T-VEC introduce tumor-suppressive genes (e.g., p53) or lyse tumors directly, providing novel cancer therapies. - Combination Strategies: Oncolytic viruses enhance immune checkpoint inhibitors and CAR T cells, overcoming barriers to solid tumor treatment. - BiTEs and CAR-T Innovations: Bi-specific T-cell engagers and CAR T cells continue to revolutionize cancer immunotherapy, especially when combined with viral agents. - Emerging Concepts (Autosis): Novel cell death pathways, such as autosis induced by myxoma virus, represent the next frontier in cancer treatment.