Transmission Media and Transceivers PDF
Document Details
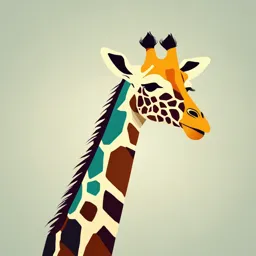
Uploaded by WellReceivedAlgebra467
Tags
Related
- COTN1220_04_Guided_And_Wireless_Media (PDF)
- 1.5 Compare and Contrast Transmission Media and Transceivers PDF
- 1.6 Compare and Contrast Transmission Media and Transceivers PDF
- Transmission Media & Wireless Technologies Study Notes PDF
- Data and Computer Communications 7th Edition PDF
- Networking Devices and Transmission Media - PDF
Summary
This document provides a review of transmission media and wireless communication technologies, including Wi-Fi standards (802.11a/b/g/n/ac/ax), cellular networks, and frequency bands. It details various types of transmission media, their characteristics, and comparisons.
Full Transcript
Transmission Media and Transceivers EXAM OBJECTIVES COVERED IN THIS SECTION 1.5 Compare and contrast transmission media and transceivers. Transmission media refers to the various channels through which data signals are transmitted between devices on a network. These media are the physical pathway...
Transmission Media and Transceivers EXAM OBJECTIVES COVERED IN THIS SECTION 1.5 Compare and contrast transmission media and transceivers. Transmission media refers to the various channels through which data signals are transmitted between devices on a network. These media are the physical pathways that connect computers, switches, routers, and other network devices, enabling communication and data exchange. Transmission media can be broadly classified into guided (wired) and unguided (wireless) media. Guided (wired) media involves physical cables that guide the data signals along a specific path. Common types of guided media include twisted pair cable, coaxial cable, and fiber optic cable. Unguided (wireless) media transmits data signals through the air or space without using physical conductors. Common types of unguided media include radio waves, microwaves, and infrared. Each type of transmission medium has unique characteristics, advantages, and limitations, which influence its suitability for different networking environments and applications. Wireless Transmission Wireless transmission media, or unguided media, allow data to be transmitted without physical connections, using electromagnetic waves to facilitate communication. Important wireless transmission media include the 802.11 standards for Wi-Fi networks, cellular networks that enable mobile communication and internet access, and satellite communication systems that provide connectivity over vast distances, including remote and rural areas. 802.11 Standards The 802.11 standards, created by the Institute of Electrical and Electronics Engineers (IEEE), are the guidelines for wireless local area network (WLAN) communication. These standards define the protocols and technologies for wireless networking, ensuring that different devices work together seamlessly. The 802.11 family covers frequency bands, data rates, modulation techniques, and security protocols. By following these standards, manufacturers can produce wireless networking equipment that functions well in various settings, whether a home network, a large enterprise, or a public Wi-Fi hotspot. Each new version of the 802.11 standards brings speed, range, reliability, and security improvements to meet the growing demand for wireless connectivity. Wireless radio technology is essential for transmitting and receiving data over the air through electromagnetic waves. These radios are the core components of wireless communication systems, enabling device communication without physical connections. By converting digital data into radio waves, wireless radios transmit these waves through the air to be received by another radio, which then converts them back into digital data. This technology is ubiquitous in modern communication systems, including Wi-Fi, Bluetooth, cellular networks, and satellite communications, offering unparalleled flexibility and mobility. Wireless frequency refers to the specific rate at which electromagnetic waves oscillate during transmission. This rate, measured in Hertz (Hz), determines the wave\'s characteristics, such as its ability to penetrate obstacles, range, and data-carrying capacity. Different wireless communication technologies use different frequencies, balancing signal range, data rate, and interference. Lower frequencies generally offer longer ranges and better penetration through obstacles, while higher frequencies can transmit more data over shorter distances. A wireless frequency band is a defined range of frequencies used for transmitting radio waves. Regulatory bodies govern these bands to ensure efficient and interference-free spectrum use. Each band is designated for specific communication types, such as Wi-Fi, cellular networks, and satellite communications. Wi-Fi commonly operates in the 2.4 GHz and 5 GHz bands, each with different trade-offs regarding range, speed, and interference. Additionally, the 6 GHz band has been introduced in Wi-Fi 6E, offering even higher speeds and reduced congestion due to more available channels. The choice of a frequency band significantly impacts the performance and suitability of a wireless communication system for various applications. In wireless communication, a channel is a smaller section within a frequency band set aside for a specific communication path. Channels let multiple devices communicate simultaneously within the same frequency band without interfering with each other. Each channel has its center frequency and bandwidth. Choosing and managing channels effectively is vital to optimizing network performance, minimizing interference, and ensuring reliable communication, especially in environments with many wireless devices. 2.4 GHz Wi-Fi Band The 2.4 GHz Wi-Fi band is one of the most popular frequency bands for wireless communication. It has a longer range and can penetrate obstacles like walls and furniture, making it perfect for home and small office setups. However, the 2.4 GHz band is often crowded with various devices like microwaves, Bluetooth gadgets, and cordless phones, which can cause a lot of interference. This band supports 14 channels, but because some channels overlap, it can lead to congestion and reduced performance. Despite these drawbacks, the 2.4 GHz band is still widely used because it can cover larger areas with fewer access points. 5 GHz Wi-Fi Band The 5 GHz Wi-Fi band became popular over the 2.4 GHz band because it has more channels and experiences less interference from commonly found household devices. This band provides faster data rates and better performance, making it attractive for tasks such as streaming videos and online gaming. It offers up to 24 channels that don\'t overlap, helping to reduce network congestion and improve overall performance. Due to the fact it uses a higher frequency, the signals can be more easily blocked by walls and other obstacles, resulting in a shorter transmission range. In these instances, additional access points will be necessary to provide the same coverage as 2.4 GHz. The 5 GHz band excels in environments where high speed and reduced interference are most important. 6 GHz Wi-Fi Band The 6 GHz Wi-Fi band, introduced with Wi-Fi 6E, is a big step forward in wireless communication. It offers faster speeds and more capacity because it has more spectrum and wider channels. With 59 non-overlapping channels, it cuts down on congestion and interference, making for a smoother and more efficient connection. The higher frequency means faster data transfer rates, which is great for things that need a lot of bandwidth and low latency, like virtual reality and large file transfers. However, similar to the 5 GHz band, the 6 GHz signals don\'t travel as far and are more easily blocked by obstacles. This band is instrumental in densely populated areas and places where devices compete for bandwidth. 802.11a Standard The 802.11a standard was one of the earliest wireless standards officially ratified in 1999. Operating in the 5 GHz frequency band, 802.11a has a maximum theoretical data transfer rate of 54 Mbps. This standard utilizes orthogonal frequency-division multiplexing (OFDM) to efficiently transmit data, reducing interference and improving performance in environments with numerous wireless devices. While its higher frequency allows for faster data transmission, it also results in a shorter range than standards operating in the 2.4 GHz band. The 802.11a standard is particularly suited for enterprise networks and environments where minimizing interference is crucial. 802.11b Standard The 802.11b standard, ratified in 1999, operates in the 2.4 GHz frequency band and supports a maximum theoretical data rate of up to 11 Mbps. Utilizing direct-sequence spread spectrum (DSSS) technology, 802.11b offers reliable performance but is more susceptible to interference from other devices operating in the same frequency range, such as microwaves and Bluetooth. Despite its slower speeds and potential for interference, 802.11b was widely adopted due to its cost-effectiveness and sufficient performance for early wireless networking needs. 802.11g Standard The 802.11g standard, ratified in 2003, was designed to overcome the shortcomings of its predecessors. It operates in the 2.4 GHz frequency band and achieves theoretical data rates up to 54 Mbps. By employing orthogonal frequency-division multiplexing (OFDM) or direct-sequence spread spectrum (DSSS), 802.11g combines the best features of 802.11a and 802.11b. This standard is backward compatible with 802.11b devices, ensuring seamless integration and improved speeds in existing networks. The 802.11g standard became a popular choice for both home and enterprise wireless networks due to its balance of speed, range, and compatibility. 802.11n Standard The 802.11n standard represents a significant leap in wireless networking technology, supporting the 2.4 GHz and 5 GHz frequency bands and offering theoretical data rates of 600 Mbps. Key to its performance are technologies such as multiple input multiple output (MIMO) and channel bonding, which enhance data throughput and range. Backward compatible with 802.11a/b/g, 802.11n can integrate with older devices while experiencing improvement in speed and reliability. This standard became a cornerstone of modern wireless networks, suitable for high-demand applications such as video streaming and online gaming. The 802.11n standard was ratified in 2009. 802.11ac Standard While advancing wireless performance, the 802.11ac standard operates exclusively in the 5 GHz frequency band and delivers data rates up to 1.3 Gbps (Wave 1) and up to 3.47 Gbps (Wave 2). Ratified in 2013, it employs wider channels, advanced modulation techniques, and multi-user MIMO (MU-MIMO) to achieve these high speeds. The 802.11ac standard is backward compatible with 802.11a/n devices, ensuring smooth transitions and enhanced performance for modern applications requiring high bandwidth, such as 4K video streaming and large file transfers. 802.11ax Standard The 802.11ax standard (Wi-Fi 6) operates in the 2.4 and 5 GHz bands, with an additional option for the 6 GHz band in Wi-Fi 6E. This standard significantly enhances efficiency and performance, with potential data rates reaching 9.6 Gbps. It is backward compatible with earlier 802.11 standards, meeting the increasing need for fast, reliable wireless connections in residential and commercial environments. Wi-Fi 6 was ratified in 2019, and Wi-Fi 6E in 2020. IEEE 802.11 Wireless (Wi-Fi) Standards. Cellular Wireless Cellular wireless communication allows mobile devices to connect to the internet and make voice calls by transmitting data through a cell tower network. This technology provides mobile connectivity across large cities, rural regions, and entire countries. Mobile Network Operators (MNOs), also known as wireless carriers or telecom providers, manage cellular radio architectures. These operators build, maintain, and manage the infrastructure for cellular communication, including cell towers, base stations, and the core network. MNOs offer services such as voice calling, text messaging, and mobile internet access to their subscribers. Cellular technology has evolved through several generations, each bringing significant improvements in speed, capacity, and capabilities. The generations of cellular technology before 4G brought significant advancements and widespread impacts. 1G introduced basic mobile voice communication but faced limitations in security, voice quality, and accessibility. The transition to 2G brought digital technology, enhancing security and voice quality while introducing SMS, making mobile phones more accessible, and fostering economic growth. The arrival of 3G revolutionized mobile communication by enabling mobile internet access and multimedia services, leading to the proliferation of smartphones and global connectivity. These advancements transformed social interactions and daily life, stimulated economic development, and set the stage for the high-speed, data-driven capabilities of 4G and beyond. 4G, or Fourth Generation cellular technology, introduced in the late 2000s, brought significant improvements in mobile communication with the adoption of LTE (Long Term Evolution) technology. It offers high-speed internet access, HD video streaming, and VoIP (Voice over IP) capabilities, providing speeds ranging from 100 Mbps to 1 Gbps. 4G greatly enhanced the reliability and speed of mobile networks, enabling more advanced applications and services. 5G, or Fifth Generation cellular technology, emerged in the late 2010s, marked by the introduction of NR (New Radio) technology. 5G offers ultra-fast internet speeds up to 10 Gbps, low latency, and massive device connectivity. It is ideal for IoT (Internet of Things), autonomous vehicles, smart cities, and real-time applications like virtual reality. It supports a wide range of new use cases and promises to revolutionize mobile connectivity with its advanced capabilities. Satellite Wireless Satellite communication is a wireless transmission media that sends and receives data signals via satellites orbiting the Earth. This technology provides connectivity in remote and underserved areas where traditional wired or ground-based (terrestrial) wireless networks are not feasible. Satellite communication works through a process known as uplink and downlink: data is transmitted from a ground station (uplink) to a satellite in space, which then relays the signal back to another ground station or user terminal (downlink). The satellite acts as a repeater, amplifying and retransmitting the signal to cover great distances. There are two primary types of satellites: geostationary satellites and low Earth orbit (LEO) satellites. Geostationary satellites are positioned about 22,236 miles above the Earth's equator and maintain a fixed position relative to the Earth\'s surface. They are perfect for broadcast services and long-distance communication. On the other hand, LEO satellites orbit at much lower altitudes (between 311 and 1,242 miles) and offer lower latency and faster data speeds compared to geostationary satellites. LEO satellites are part of large constellations to ensure continuous global coverage. Satellite communication has several advantages, including global coverage, reliability, and broadcast capabilities. Satellites can connect virtually any location on Earth, including remote and rural areas, oceans, and mountains. Satellite networks are less susceptible to terrestrial disruptions such as natural disasters or infrastructure damage. Additionally, they are ideal for broadcasting services like television and radio, where the same signal needs to be distributed over a wide area. Satellite communication has limitations. Geostationary satellites have a much higher latency (approximately 500 - 700 milliseconds) compared to satellites. This is due to the long distance signals must travel. The monetary investment in launching and maintaining satellites is considerable, resulting in increased costs passed on to customers. Unfavorable weather conditions, such as snow, rain, or storms, can have an impact on signal quality and reliability. Applications of satellite communication are varied and include broadband internet services in remote and rural areas, distribution of television and radio broadcast signals, support for global positioning systems (GPS) for navigation, ensuring communication capabilities in disaster-affected areas, and offering secure communication for military operations and strategic applications. Wired Transmission Wired transmission media, or guided media, refers to the physical cables used to transmit data signals in a network. These media include various types of cables, each with specific characteristics and applications. Key topics under wired transmission media include the 802.3 standards for Ethernet networking, the differences between single-mode and multimode fiber optics, the use of Direct Attach Copper (DAC) cables, including twinaxial cables, and the properties and applications of coaxial cables. Additionally, understanding cable speeds and the distinction between plenum and non-plenum cables is crucial for designing and maintaining efficient and safe wired networks. 802.3 Standards The IEEE developed the 802.3 standards to define the specifications for Ethernet networking. These standards cover various aspects of Ethernet, including physical layer specifications, data rates, and media types. The 802.3 standards ensure interoperability and compatibility among different network devices and provide guidelines for implementing reliable and high-speed wired networks. Understanding these standards is essential for designing, deploying, and managing Ethernet networks in residential and commercial environments, supporting a range of applications from basic Internet access to complex enterprise solutions. xBASE-y Ethernet Naming Convention The xBASE-y naming convention describes various Ethernet standards and provides information about the technology\'s data transmission speed, the type of transmission, and the physical medium used. This convention describes: x - This part of the name specifies the data transmission speed of the Ethernet standard. The number is typically given in megabits per second (Mbps) or gigabits per second (Gbps). For example: 10BASE-T10 Mbps 100BASE-TX100 Mbps 1000BASE-T1000 Mbps (1 Gbps) 10GBASE-T10 Gbps (10000 Mbps) BASE - The term \"BASE\" indicates that the Ethernet standard uses baseband transmission. Baseband transmission means that the entire bandwidth of the cable is used for a single data channel, as opposed to broadband transmission, which can carry multiple signals on different frequencies. y - The final part of the name specifies the physical medium (or media type) used for the Ethernet standard and sometimes includes information about the maximum segment length. Common notations include: T: Twisted pair cabling TX: Twisted pair cabling (with additional specifications) SX: Short-wavelength laser over multimode fiber LX: Long-wavelength laser over single-mode fiber SR: Short range over multimode fiber LR: Long range over single-mode fiber For example, 1000Base-T denotes an Ethernet implementation that operates at a maximum data transfer rate of 1000 Mbps (1000), uses baseband signal transmission (BASE), and runs over twisted pair cabling (-T). Twisted Pair Cables Twisted pair copper cables are widely used for data transmission in Ethernet networks. These cables are popular due to their cost-effectiveness, ease of installation, and reliable performance. Copper cables transmit data through electrical signals that travel along the copper conductors. As the signals travel, they can experience attenuation, or signal loss, over distance, reducing the effectiveness and speed of data transmission. Twisted Pair Category Ratings Category ratings, often referred to as CAT ratings, are specifications for twisted pair cables used in Ethernet networks. These ratings define the cable\'s performance characteristics, such as maximum data transmission speed, frequency, and shielding. Different Ethernet standards require specific Category cables to ensure reliable and efficient data transmission. Category ratings for twisted pair cables were developed by the American National Standards Institute (ANSI), the Telecommunications Industry Association (TIA), and the Electronic Industries Alliance (EIA). The TIA and EIA work together to create and publish standards for telecommunications and electronic equipment, including structured cabling systems used in networking. ANSI TIA/EIA category standards for twisted pair cabling. IEEE 802.3 Specifications for Copper Twisted Pair Cabling The IEEE 802.3 standards encompass a wide range of Ethernet networking technologies. The following is an overview of some key 802.3 standards for copper twisted pair cabling and their characteristics: IEEE 802.3 Ethernet Standards for twisted pair copper cable. 10BASE-T and 100BASE-TX 10BASE-T and 100BASE-TX are two legacy Ethernet standards that played significant roles in the development of local area networks (LANs). 10BASE-T was one of the earliest Ethernet standards and is now largely obsolete, having been replaced by faster technologies. On the other hand, 100BASE-TX, or Fast Ethernet, provided a significant speed boost over 10BASE-T and was widely used in network upgrades. However, it has largely been superseded by Gigabit Ethernet (1000BASE-T) in modern network installations. 1000BASE-T 1000BASE-T, commonly known as Gigabit Ethernet, represents a significant advancement in Ethernet technology, providing much faster data transmission compared to its predecessors. This standard allows for seamless integration into existing Ethernet networks, offering a cost-effective upgrade path from older technologies like 10BASE-T and 100BASE-TX. By enhancing network performance and supporting a wide range of applications, 1000BASE-T has become the standard for modern networking. 40GBASE-T 40GBASE-T is an advanced Ethernet standard designed to provide ultra-high-speed data transmission, significantly enhancing network performance. It was developed to address the needs of data centers and enterprise networks. This increase in performance supports very high bandwidth requirements, enabling faster data processing, storage access, and virtualization. This standard offers a scalable and efficient upgrade path from lower-speed Ethernet technologies, ensuring compatibility with existing network infrastructure while delivering superior performance. Fiber Optic Cables Fiber optic cables implemented for Ethernet communication primarily operate in the infrared light spectrum. Light signals travel through the core of the fiber optic cable, reflecting off the cladding to keep the signal contained within the cable. This method allows data transmission over much longer distances without significant signal loss. Surrounding the cladding is the buffer, which provides an additional protective layer for the delicate core. The strength member offers further strength and protection and is designed to withstand the stress of cable installation. Basic construction of a single-core fiber optic cable. Despite their efficiency, fiber optic cables can still experience attenuation, especially over very long distances. To mitigate this, repeaters or amplifiers boost the signal and maintain data integrity over extended lengths. The advantages of fiber optic cable (compared to copper cable) include higher bandwidth, longer distances, immunity to electromagnetic interference, and higher security (it is more difficult to tap without detection). On the other hand, the disadvantages of fiber optic cable (compared to copper cable) include higher installation and maintenance costs, fragility, and more complex installation. Single Mode Fiber (SMF) and Multimode Fiber (MMF) Fiber optic cables are specified based on several characteristics. The mode refers to whether the fiber is Single Mode Fiber (SMF) or Multimode Fiber (MMF). SMF, with its smaller core, allows only one light mode to propagate, making it ideal for long-distance and high-bandwidth applications. In contrast, MMF supports multiple light modes, which are suitable for shorter distances and high-speed data transfer. Fiber optic cables use glass or plastic fibers for the core, and glass fibers are preferred for high-performance and long-distance communication due to their lower attenuation and higher bandwidth capabilities. Plastic fibers are used for short-distance and cost-sensitive applications. Single mode fiber (SMF) cables typically have a core diameter of 8-10 micrometers, with a cladding diameter of 125 micrometers. It typically uses infrared wavelengths of 1310 nm and 1550 nm. MMF cables have larger core diameters, usually 50 or 62.5 micrometers, with a cladding diameter of 125 micrometers. It operates at the 850 nm and 1310 nm infrared wavelengths. Core and cladding diameter comparison. IEEE 802.3 Specifications for Fiber Optic Cabling The IEEE 802.3 standards include the use of fiber optic cabling. The following is an overview of some key 802.3 standards for fiber optic cabling and their characteristics: IEEE 802.3 Ethernet Standards for fiber optic cable. 100BASE-SX, 100BASE-FX 100BASE-SX is designed for short-distance, high-speed networking within buildings, typically using multimode fiber and operating at an 850 nm wavelength. While it was once a popular choice for Fast Ethernet connections in local area networks (LANs), its use has significantly declined with the widespread adoption of Gigabit Ethernet (1000BASE-SX) and higher-speed standards. Today, 100BASE-SX is largely considered obsolete and is rarely used in new installations. 100BASE-FX, designed for longer distances and using a 1300 nm wavelength with both multimode and single-mode fibers, was also widely used for extending network connections across campuses and between buildings. Like 100BASE-SX, its use has declined as faster Ethernet standards, such as 1000BASE-FX and 10 Gigabit Ethernet, have become more common. Both of these standards may still be found in some legacy systems where upgrading to higher speeds is not necessary or cost-effective. 1000BASE-SX, 1000BASE-LX 1000BASE-SX and 1000BASE-FX are both standards for Gigabit Ethernet over fiber optic cables, each suited to different needs. 1000BASE-SX is great for short distances, like within buildings and data centers, using multimode fiber and operating with an 850 nm wavelength. It\'s a cost-effective choice for high-speed networking in these environments. On the other hand, 1000BASE-FX is designed for longer distances, using a 1300 nm wavelength and working with both multimode and single-mode fibers. It\'s ideal for connecting different buildings on a campus or other scenarios where you need a reliable connection over a greater distance. 10GBASE-SR, 10GBASE-LR 10GBASE-SR and 10GBASE-LR are both standards for 10 Gigabit Ethernet, each suited for different networking scenarios. 10GBASE-SR is designed for short-range applications, using multimode fiber and operating at an 850 nm wavelength. It is ideal for high-speed connections within data centers and enterprise environments, supporting distances up to 400 meters. On the other hand, 10GBASE-LR is intended for long-range applications, utilizing single-mode fiber and operating at a 1310 nm wavelength. It supports distances up to 10 kilometers, making it suitable for connecting data centers, campuses, and metropolitan area networks. Ethernet standards higher than 40 Gbps, such as 100G, 200G, and 400G, are increasingly prevalent in data centers, enterprise networks, telecommunications, and high-performance computing environments. The demand for higher bandwidth, scalability, and performance drives this adoption, with future trends pointing toward even higher speed standards. Coaxial Cables Coaxial cable consists of a central copper or copper-clad steel conductor that carries the signal, surrounded by a dielectric insulator for spacing, a metallic shield (aluminum or copper foil/braiding) for EMI and RFI protection, and an outer jacket (PVC or polyethylene) for durability and physical protection. This design ensures efficient high-frequency signal transmission with minimal interference and robust durability. Basic construction of a coaxial cable. (Source: L-com.com) While coaxial cable has largely been replaced by twisted pair and fiber optic cables in many networking applications, it remains relevant in specific areas such as broadband internet access, television and satellite services, in-building signal distribution, and security systems. Its excellent shielding properties and durability continue to make it a valuable medium for certain modern networking and communication needs. The use of appropriate radio grade (RG) ratings, such as RG-6, ensures that coaxial cables meet the specific requirements of these applications. Direct Attach Copper (DAC) Cables Direct Attach Copper (DAC) cables are high-speed, short-range connections commonly used within data centers. They consist of twinaxial copper cables with integrated transceivers at both ends. Basic construction of a twinaxial cable. (Source: Pasternack.com) DAC cables support various Ethernet standards and speeds, including 10 Gbps (10GBASE-CU), 40 Gbps (40GBASE-CR4), and 100 Gbps (100GBASE-CR4). Ideal for distances up to 7 meters, DAC cables provide plug-and-play simplicity, low latency, and high performance for high-density networking environments, making them perfect for connecting servers to top-of-rack (ToR) switches, storage systems, and network interface cards. Direct Attach Copper (DAC) cable with 10G SFP+ transceivers. (Source: FS.com) Direct Attach Copper (DAC) cable connecting two high-speed switches. (Source: Cables-Solutions.com) Plenum versus Non-Plenum Cable A plenum is a space used for circulating air in a building\'s heating, ventilation, and air conditioning (HVAC) systems. Common plenum spaces include the areas above drop ceilings and below-raised floors, which are implemented to facilitate the movement of air. Because air flows through these spaces, any materials used within plenums, such as network cables, must meet strict fire safety standards to prevent the spread of smoke and toxic fumes in the event of a fire. (Left) Non-plenum airspace- all air movement is contained in ducts. (Right) Plenum airspace- return air movement is in open space. (Source) In networking, the terms \"plenum\" and \"non-plenum\" refer to the types of cabling used in different building environments, particularly concerning their fire resistance and safety characteristics. Plenum cables are specifically designed for use in plenum spaces. Made with fire-retardant materials that produce less smoke and fewer toxic fumes when burned, these cables meet stringent fire safety standards and are essential for preventing the spread of fire through air ducts. These characteristics make plenum cables more expensive than their non-plenum counterparts. Plenum cables are identified by the markings "CMP" and "OFNP." Non-plenum cables are used in areas where air circulation does not occur, such as within walls or between floors. These cables do not have the same fire-resistant properties and are typically made from standard PVC, making them a more cost-effective option for general use where stringent fire safety measures are not required. Non-plenum cables are identified by the markings "CMR" and "OFNR." Plenum and non-plenum cables are available in copper twisted pair, coaxial, and fiber optic forms, each designed to meet specific fire safety standards for different installation environments. Transceivers Transceivers are components in networking that enable the transmission and reception of data signals. They convert electrical signals from network devices into optical signals for transmission over fiber optic cables or vice versa. Transceivers are used in a variety of protocols and come in multiple form factors to accommodate different network requirements. Protocols Ethernet - Ethernet transceivers are used to connect network devices such as switches, routers, and servers, enabling data communication over Ethernet networks. They support various Ethernet standards, including Fast Ethernet (100 Mbps), Gigabit Ethernet (1 Gbps), 10 Gigabit Ethernet, and higher speeds. Ethernet transceivers are widely used in both local area networks (LANs) and wide area networks (WANs) for reliable and high-speed data transfer. Fibre Channel - Fibre Channel transceivers are designed for storage area networks (SANs), providing high-speed data transfer between data storage systems and servers. Fibre Channel is known for its low latency, high reliability, and ability to handle large volumes of data. Fibre Channel transceivers are primarily used in data centers for connecting storage devices. Form Factors Small Form-Factor Pluggable (SFP) - SFP transceivers are compact, hot-swappable modules that can be easily inserted into network devices. They support data rates up to 4.25 Gbps and are used for both fiber optic and copper connections. This form factor is commonly used in switches, routers, and other networking equipment for both short-range and long-range data transmission. SFPs support various standards, including Ethernet and Fibre Channel. 10 GbE, LC MMF, Small-Form Factor Pluggable Plus (SFP+) transceiver with dust cap inserted. (Source) Quad Small Form-Factor Pluggable (QSFP) - QSFP transceivers are similar to SFPs but support higher data rates, typically ranging from 4 Gbps to 100 Gbps or more. The \"quad\" designation indicates that QSFP modules can support four channels of data simultaneously. They are used in high-density data center environments for applications requiring high bandwidth, such as 40 Gigabit Ethernet (40GbE) and 100 Gigabit Ethernet (100GbE). QSFP modules are ideal for backbone and aggregation layer connections in modern networks. 40GBASE-LR4, LC SMF, Quad Small-Form Factor Pluggable Plus (QSFP+) transceiver with dust cap inserted. (Source: FS.com) Copper Connector Types RJ11 Registered jack (RJ11) connectors are typically used for twisted pair telephone lines and are commonly found in residential and office environments. They have six positions but usually use only two or four of these for wiring. They are primarily used for connecting telephones, modems, and other telecommunication devices to the telephone network. RJ45 Registered jack (RJ45) connectors are the standard for Ethernet networking and are used to connect twisted pair cables to network devices such as switches, routers, and computers. They have eight positions and use all eight for wiring, supporting various Ethernet standards from Fast Ethernet (100 Mbps) to Gigabit Ethernet (1 Gbps) and beyond. RJ45 connectors are commonly used in both residential and commercial environments for establishing wired network connections, including local area networks (LANs) and internet connectivity. Registered jacks. (Source: UNC Group) Bayonet Neill-Concelman (BNC) BNC (Bayonet Neill--Concelman) connectors are used to connect and disconnect coaxial cables quickly. They feature a bayonet mount mechanism that provides a secure connection. BNC connectors are implemented in professional video and radio frequency (RF) applications, including television broadcasting, military equipment, and test instruments. F-Type F-Type connectors are threaded connectors commonly used with coaxial cables for television and cable internet connections. They provide a reliable and secure connection through a screw-on design. These coaxial connectors are primarily used in residential and commercial applications for connecting cable television, satellite television, and cable modems. Coaxial cable connectors. (Source: BNC, F-Type) Fiber Optic Connector Types Fiber optic cable connectors. SC and SC have dust caps inserted, and LC and MPO have dust caps removed. (Source: FS.com) Subscriber Connector (SC) SC connectors are square-shaped, push-pull connectors known for their ease of use and reliability. They feature a simple push-in and pull-out mechanism, making them convenient for quick connections. It can be used for single-mode and multimode fiber optic cables. SC connectors are often used with Gigabit Ethernet and 10 Gigabit Ethernet. Lucent Connector (LC) LC connectors are smaller, compact connectors with a push-pull design similar to SC connectors. They have a latch mechanism for secure connections and are higher density, making them ideal for high-density applications. LC connectors are used with both single-mode and multimode fiber optic cables and are implemented for Gigabit Ethernet and 10 Gigabit Ethernet. Straight Tip (ST) ST connectors are round, bayonet-style connectors that require a twist and lock mechanism. They are one of the oldest types of fiber optic connectors and are known for their ruggedness. They are primarily used with multimode fiber optic cables but can also be used with single-mode fibers. The ST connector is considered a legacy option, primarily due to its older design and the shift towards more compact and higher-density connectors like LC. Historically, this connector was used for Fast Ethernet and Gigabit Ethernet implementations. Multi-Fiber Push-On MPO) The MPO (Multi-Fiber Push-On) connector is a high-density fiber optic connector extensively used in modern networking, especially in applications requiring high bandwidth and efficient space utilization. It is designed to handle multiple optical fibers, typically 12 or 24, within a single rectangular ferrule, enabling dense connections. The MPO connector, featuring a push-pull latching mechanism, allows for easy insertion and removal. It is commonly used in 40 Gigabit Ethernet and 100 Gigabit Ethernet applications. MPO connectors are prevalent in data centers, high-speed networks, and environments where space is limited and high bandwidth is critical. They are often used for backbone and horizontal cabling, as well as in parallel optics applications. Network Topologies, Architectures, and Types Completion requirements View 1.6 Compare and contrast network topologies, architectures, and types. A network is two or more devices or systems connected together through some type of network transmission medium and configured with one or more protocols that enable them to exchange information. Illustration of a network topology comparing houses, driveways, and streets to networked devices, transmission medium, and connectivity devices. A network can be defined by its size and scope. The size of a network refers to the number of devices (nodes) connected within it, the physical area it covers, and its overall capacity to handle data. The scope of a network describes how far it can reach geographically and how extensive its operations are. It defines the network\'s purpose, what it can do, and the type of technology it uses. Network architecture defines the overall design and structure of a network, encompassing both its physical and logical components. It includes the arrangement of hardware, software, communication protocols, and transmission media used to establish, manage, and secure data communications. For example, a client-server architecture utilizes centralized servers that provide resources and services to client devices. In a Peer-to-Peer (P2P) architecture, devices communicate directly with each other without a centralized server. Network types categorize networks based on their scale, purpose, and geographical reach. This classification helps to identify the suitable network design and technology for specific needs. For example, a LAN type covers a small geographic area and provides high-speed connectivity within that area. A WAN type spans large geographical areas, connects multiple LANs, and enables long-distance communication and data sharing. Two prevalent network types include the following: A local area network (LAN) interconnects computers and other devices within a limited geographical area. It allows for low latency, high-speed data transfer, and resource sharing among connected devices. A LAN is commonly owned and managed by a single organization or individual and uses wired connections like Ethernet cables or wireless technologies such as Wi-Fi. The geographical scope of a LAN limits its implementation to a home, office, or small group of buildings. A wide area network (WAN) is a network that spans large geographical areas, often connecting multiple local area networks (LANs) across cities, states, or countries. It is designed to enable long-distance communication and data sharing between remote locations and is essential for organizations with multiple branches or global locations. WANs typically utilize various transmission media such as fiber optics, satellite links, and leased lines, resulting in higher latency and generally lower data transfer rates compared to LANs. WANs are managed by multiple organizations or service providers. The internet is the most extensive example of a WAN, connecting millions of networks worldwide. Network Topology Network topology describes the layout and connections of devices within a network, such as how nodes are linked and communicate with each other. For example, in a wired star topology, all devices are connected to a central connectivity device via a dedicated network cable. In a mesh topology, each device is interconnected with multiple other devices. A network topology can be described in terms of its physical or logical layout. Physical network topology refers to the actual layout and arrangement of network devices, cables, and other hardware components in a network. It illustrates how devices are physically connected to each other and where they are located. This includes showing the physical paths that data takes, the type of cables, and the hardware used. For example, in a star topology, devices are physically connected to a central hub or switch, whereas in a bus topology, all devices connect to a single central cable or backbone. Logical network topology describes the way data flows within a network, regardless of its actual physical design. It defines the paths that data takes between devices and how they communicate logically. This focuses on the logical paths that data packets follow, which may differ from the physical connections, and emphasizes network protocols and data exchange processes. The key differences between the two are in their focus and representation. Physical topology is concerned with the tangible connections and hardware layout, focusing on installation and cabling, while logical topology deals with the data flow and communication paths, focusing on network protocols and data routing. Point-to-Point Topology Point-to-point topology is a simple network design where a direct link connects two network devices, such as computers, switches, or routers. This type of connection provides a dedicated communication channel between the two devices. A point-to-point link can be a physical topology (OSI layer 1) or a logical topology (OSI layer 2, 3). In terms of physical topology, a point-to-point link involves a direct physical connection between two devices using a specific medium, such as a cable (e.g., Ethernet, fiber optic) or a wireless connection (e.g., microwave link). This physical layout shows the actual pathway that data travels between the two devices. A true point-to-point link typically does not include intermediate devices or networks. The physical connection would be a direct cable or wireless link between the two devices without passing through other networks. In terms of logical topology, a point-to-point link represents the direct data flow and communication path between two devices, regardless of the physical connection. This logical perspective focuses on how data is transmitted between the devices, ensuring that the communication is exclusive and dedicated. The communication path between two devices is considered direct in terms of data flow, even if the data passes through several intermediate devices or networks. For instance, in a VPN connection, two devices may establish a logical point-to-point connection over the internet, even though the data travels through numerous routers, switches, and other networks in between. (Top) Physical point-to-point link indicated by solid line. (Bottom) Logical point-to-point link indicated by dashed line. The cloud represents unspecified intermediate networks or devices. Star Topology A physical star topology is a network configuration where all devices (nodes) are connected to a central connectivity device, such as a switch. Each device has a dedicated cable connecting it directly to the switch, forming a star-like pattern. None of the networked nodes are directly connected. The physical star topology simplifies network management and troubleshooting, as issues can be isolated to individual devices or cables. The topology also offers scalability, as adding new devices is straightforward, requiring only a direct connection to the switch. However, the central switch is a single point of failure; if it fails, the entire network is affected. Physical star topology example using a switch as central connectivity device. Hub and Spoke Topology Hub-and-spoke topology is a network configuration where all devices (spokes) are connected to a central device (hub). The hub serves as the main connection point, facilitating data transfer between the spokes. All traffic from spoke to spoke must pass through the hub. This topology is scalable, however, the hub is a single point of failure and a potential bottleneck. It resembles a star topology but is commonly used in WANs and large-scale networks where central management is required. Hub and spoke topology example. All traffic between spokes must pass through the hub. Mesh Topology Mesh topology is a network configuration where each device (node) is interconnected with multiple other devices, creating a web-like structure. This topology can be implemented as either a full mesh or partial mesh. Full mesh means every device is connected to every other device. Conversely, a partial mesh is where some devices are connected to all others, while some are connected only to those with which they need to communicate. The high redundancy of mesh topology provides multiple paths between any two nodes, enhancing network reliability as data can be rerouted through other paths if one link fails. One of the main advantages of mesh topology is its fault tolerance. The multiple redundant paths mean there is no single point of failure, so the network can continue to operate even if one or several links or nodes fail. Additionally, direct paths between nodes can reduce the need for data to travel through multiple hops, leading to faster transmission. However, the increased number of connections makes configuration and management more complex, especially in full mesh networks. Mesh topology does come with disadvantages, primarily the high cost due to the extensive cabling and network hardware required. The increased complexity in configuration and network management also poses a challenge, particularly as the network grows. While partial mesh topologies are more scalable, full mesh topologies can become impractical in very large networks due to the exponential increase in connections. Mesh topology is widely used in various applications. It is employed in wide area networks (WANs) and metropolitan area networks (MANs) to connect multiple sites with high reliability. In wireless networks, mesh topology ensures robust communication and coverage, as seen in wireless mesh networks. Data centers also utilize mesh topology to ensure high availability and fault tolerance between servers and storage systems. The benefits of high fault tolerance, no single point of failure, and efficient data routing make mesh topology a valuable choice for many critical networking environments. Full mesh and partial mesh topology examples. Hybrid Topology A hybrid topology is a network configuration that combines two or more different types of topologies to take advantage of the strengths and diminish the weaknesses of each individual topology. Oftentimes, a single topology will not meet the requirements of an enterprise network, campus network, or data center. The hybrid topology allows for a more flexible, scalable, and reliable network design that can be tailored to specific organizational needs. Modern hybrid network topologies have the advantage of optimized performance, reliability, and scalability. Enhanced fault tolerance, redundancy, and customization are important factors for using a hybrid topology. Hierarchical hybrid topology example. A common topology where multiple star networks are connected to a mesh network. Traffic Flows Traffic flow in a network refers to the movement of data packets between devices and systems, encompassing all data transmissions that occur within the network. This includes communication between users, applications, and services. Traffic flow involves several significant components: data packets, sources and destinations, pathways, and protocols that govern data exchange. Types of traffic flow include unicast, broadcast, and multicast. The flow of traffic is influenced by factors such as network topology, bandwidth, latency, congestion, and the efficiency of routing and switching devices. The two primary types of traffic flows are north-south and east-west. North-south traffic refers to data movement between clients and servers, typically flowing from the edge of the network (end devices) to the core (servers, data centers) and vice versa. This type of traffic flow moves vertically through the network hierarchy, passing through the access layer, distribution layer, and core layer. An example of north-south traffic could be where a user accesses a website. The request travels from the user's browser, through the network, to the server hosting the webpage. East-west traffic refers to data movement between servers and devices within the same data center or network layer, typically lateral or horizontal movement. East-west traffic moves within the same network, such as between servers within a data center. An example is data replication between two Windows AD DS servers. East-west traffic moves within the data center from server to server, while north-south traffic moves from clients to servers. Three-Tier Hierarchical Model The three-tier hierarchical model is a network design framework used to manage and organize large-scale networks efficiently. It divides the network into three distinct layers: core, distribution, and access, each serving a specific role in data handling and traffic management. Three-tier hierarchical model example. The core layer acts as the backbone of the network, providing high-speed, reliable data transfer across different parts of the network. It connects multiple distribution layers and is responsible for fast and efficient data routing. The core layer is designed to handle large volumes of data with minimal latency and is typically composed of high-capacity, high-performance routers and switches. Key features of the core layer include high speed, fault tolerance, minimal latency, and redundancy. The distribution layer serves as an intermediary between the core and access layers, aggregating data from the access layer before it is sent to the core layer and vice versa. It implements policies, routing, filtering, and quality of service (QoS). The distribution layer manages traffic coming from multiple access layers and ensures it is properly routed to its destination. Key features of the distribution layer include policy implementation, routing, load balancing, and data aggregation. The access layer is the closest layer to end users, connecting devices like computers, printers, and wireless access points to the network. It provides network access to end devices and enforces policies for user authentication and access control. Key features of the access layer include device connectivity, user access control, and initial data handling. The three-tier hierarchical model is suitable for large enterprises with multiple departments or branches, ensuring efficient data management and high performance. It is commonly used in data centers to manage vast amounts of data traffic with minimal latency and high reliability. University campuses also employ this model to connect various buildings and departments, providing an organized and scalable network infrastructure. Collapsed Core The collapsed core architecture is a simplified network design that merges the core and distribution layers of the traditional three-tier hierarchical model into a single layer. This two-tier design streamlines network structure, making it easier to design, implement, and manage, which is particularly advantageous for smaller networks. Centralizing routing and traffic management functions within the collapsed core layer reduces the need for additional hardware, cabling, and maintenance, leading to lower costs. However, this simplicity can become a limitation in larger networks, as it may not scale as efficiently and can create a single point of failure if redundancy is not implemented. Collapsed core architecture example. Spine and Leaf Topology Spine and leaf topology is a network architecture commonly used in modern data centers to optimize performance, scalability, and fault tolerance. It consists of two main layers: the spine layer and the leaf layer. The spine layer in a spine and leaf topology consists of high-capacity core switches that form the backbone of the network. Each spine switch is connected to every leaf switch, ensuring uniform connectivity and multiple paths for data transmission. This reduces the risk of bottlenecks and ensures consistent performance across the network. The architecture allows for easy scalability. New spine switches can be added to increase the network\'s overall capacity without disrupting the existing setup. The redundant connections between the spine and leaf switches provide fault tolerance. If one spine switch fails, the leaf switches can route traffic through other spine switches, maintaining network continuity. The leaf layer consists of access switches that connect directly to servers, storage devices, and other network endpoints. Each leaf switch is connected to every spine switch, creating a non-blocking architecture with multiple paths for data. New leaf switches can be added to accommodate additional devices or increase network capacity without major reconfiguration. This makes it easier to scale the network to meet growing demands. The leaf layer is primarily responsible for handling east-west traffic, which refers to data exchanges within the data center, such as between servers. Spine and leaf topology diagram. Note the spine layer- no spine switches are connected together. Note the leaf layer- no leaf switches are connected together, and each switch is configured in a mesh with the spine switches. Summary In this chapter, we covered the fundamentals of network transmission media, transceivers, and topologies. We explored the characteristics and applications of both wired and wireless transmission methods, including various standards and cable types. Additionally, we discussed different network topologies and architectures, highlighting their design principles and the flow of data within network structures. At the completion of this chapter, you should be able to: Compare and contrast transmission media and transceivers. Compare and contrast network topologies, architectures, and types. In this chapter, we covered: Transmission Media and Transceivers Network Topologies, Architectures, and Types IPv4 Network Addressing Completion requirements View EXAM OBJECTIVES COVERED IN THIS SECTION 1.7 Given a scenario, use appropriate IPv4 network addressing. IPv4 network addressing is a fundamental aspect of networking that involves assigning unique addresses to devices on a network and ensuring that data packets are accurately routed to their intended destinations. Understanding IPv4 addressing is crucial for network design, implementation, and troubleshooting. This section will explore key concepts and practices related to IPv4 addressing, including the distinction between public and private addresses, the use of Automatic Private IP Addressing (APIPA), and the functionality of loopback addresses. Additionally, we will examine subnetting, a critical technique for dividing more extensive networks into smaller, manageable sub-networks to optimize performance and security. Network Addressing To understand network addresses, you must understand how base numeral systems work. For example, consider a familiar numeral system: the base ten numeral system, also known as the decimal system. The base ten numeral system is a positional numeral system that uses ten digits (0-9). Each digit\'s position represents a power of 10, with the rightmost digit representing 100, the next digit to the left representing 101, and so on. The following table is a simple review showing each digit\'s positional values and expanded forms in the decimal number 4902. It also summarizes these values to illustrate how the number is constructed. Binary (Base 2) Numeral System To expand our understanding of numeral systems, let\'s explore the base two numeral system, commonly known as the binary system. Unlike the base ten system, the binary system uses only two digits: 0 and 1. This system is crucial in computing and digital electronics, underpinning how data and instructions are represented and processed. In the binary system, each digit\'s position corresponds to a power of 2, with the rightmost digit representing 20, the next digit to the left representing 21, and so forth. The following table reviews each digit\'s positional values and expanded forms in the binary number 1011. It demonstrates the conversion of this binary number to its decimal equivalent. What does the table look like for an 8-bit binary number? Given a binary value of 10111101, convert it to decimal. In the previous examples, we converted a binary number to a decimal. How are decimal numbers converted to binary? The following method illustrates one of many ways. Given the decimal value of 120: Can 128 be subtracted from 120, resulting in a zero or positive remainder? ANSWER = No. Put a zero in the 128s place. Continue. Next, move to 64's place. Can 64 be subtracted from 120, resulting in a zero or positive remainder? ANSWER = Yes. Put a one in the 64s place. Subtract 120 -- 64 to get a remainder, = 56 Next, move to 32's place. Can 32 be subtracted from 56, resulting in a zero or positive remainder? ANSWER = Yes. Put a one in the 32s place. Subtract 56 - 32 to get a remainder, = 24 Next, move to 16's place. Can 16 be subtracted from 24, resulting in a zero or positive remainder? ANSWER = Yes. Put a one in the 16s place. Subtract 24 - 16 to get a remainder, = 8 Next, move to 8's place. Can 8 be subtracted from 8, resulting in a zero or positive remainder? ANSWER = Yes. Put a one in the 8s place. Subtract 8 -- 8 to get a remainder, = 0 The conversion process completes when the remainder equals zero. However, there are three places with empty binary values. Fill the remaining places to the right with padding (zeros). The completed 8-bit binary equivalent of decimal 120 is 01111000, read from left to right. This number is not a decimal 1,111,000 (one million, one hundred eleven thousand) but zero, one, one, one, zero, zero, zero. Note that an 8-bit binary number must always have precisely eight digits, which includes leading zeros. Ensuring an 8-bit binary number has eight digits allows for standardized representation and avoids confusion between binary values. Likewise, a 4-bit binary number must always have exactly four digits, including leading zeros. Maintaining the correct number of digits ensures that binary values such as 0100, 0010, and 0001 are distinct and correctly represent the numbers 4, 2, and 1 in decimal, respectively. For example, converting the decimal number 120 to binary without proper padding could result in ambiguity (e.g., 01111 could be confused with decimal 15). Hexadecimal (Base 16) Numeral System Let\'s explore the hexadecimal numeral system, often called base 16, to expand our understanding of numeral systems further. This system is advantageous in computing and digital electronics because it can represent large binary numbers more compactly. The hexadecimal system uses sixteen distinct symbols: 0 to 9, which represent decimal values zero to nine, and A to F, which represent ten to fifteen. As you can see in the previous table, each hexadecimal digit is four bits long. In other words, it takes four binary bits to represent each hexadecimal digit. Consider a more extended, 8-bit hexadecimal number, AF. What is the binary equivalent of the hexadecimal number AF? Given a hexadecimal value of AF: Break the hexadecimal number into 4-bit parts. (Remember, each hexadecimal digit is 4-bits long.) Convert each hex digit to decimal. Convert the decimal value for each hex digit into binary. We now have the binary equivalent of hexadecimal AF. Read from left to right; the binary value is 10101111. On the other hand, to convert from 8-bit binary 10101111 to hexadecimal: Break the binary value into 4-bit parts. Convert each 4-bit part into decimal. Convert each decimal part into hexadecimal. We now have the hex equivalent of binary 10101111. Read from left to right; the hex value is AF (sometimes written as 0xAF). Physical Network Addressing Physical network addressing refers to the hardware-level identification used in networking to distinguish individual devices on a network. This type of addressing involves the Media Access Control (MAC) address, a network segment-unique identifier for communications at the data link layer (Layer 2). A MAC address is a 48-bit identifier usually represented as six pairs of hexadecimal digits, such as 00:1A:2B:3C:4D:5E. It always contains twelve hexadecimal digits and may or may not use groups of digits or separators. For example, the following eight values all represent the same MAC address. A valid MAC address is a string of twelve digits expressed in the context of a MAC address and denoted using hexadecimal (0 to 9, A to F). A MAC address is also called an Ethernet address, physical address, hardware address, or Layer 2 address. Each MAC address assigned to a network interface card (NIC) is unique. Unique MAC addresses guarantee that no two devices on the same local network have the same MAC address, which is accomplished through an OUI. The MAC address is divided into two parts: the Organizationally Unique Identifier (OUI), which is the first six hexadecimal digits (24 bits or 3 bytes) representing the manufacturer of the NIC, and the NIC Specific part, which are the remaining six hexadecimal digits (24 bits or 3 bytes) unique to each NIC produced by the manufacturer. The MAC address 001A2B3C4D5E has an OUI of 001A2B, and if we use an OUI lookup tool, we determine this OUI has been assigned to Ayecom Technology Co., Ltd. The remaining 24 bits, 3C4D5E, have been uniquely assigned only to this specific NIC. In Ethernet networks, each Layer 2 frame includes a destination MAC address and a source MAC address in its header, ensuring that frames will be delivered to the correct device within the local network. The Address Resolution Protocol (ARP) maps an IP address to a MAC address, allowing communication within a local network segment. When a device needs to communicate with another on the same network segment, it sends an ARP request to find the MAC address linked to the target IP address. The target device responds with an ARP reply containing its MAC address. A Layer 2 broadcast domain is a network segment in which any broadcast packet sent by a device is received by all other devices within the same segment. This occurs because all devices in the broadcast domain share the same network Layer 2 infrastructure, typically connected through switches or bridges. In a Layer 2 network, when a device wants to send a broadcast message, it sends a frame to the special broadcast MAC address (FF:FF:FF:FF:FF). This frame is then propagated to all ports on the switch except the one it originated from. Every device in the same broadcast domain receives and processes the broadcast frame. A Layer 2 broadcast domain with hosts connected via a Layer 2 switch. A Layer 2 broadcast domain is extended with the addition of Layer 2 switches. Logical Network Addressing Logical network addressing is used to identify devices and networks at a higher level, allowing for efficient routing and communication across interconnected networks. Unlike physical addresses, which are hardware-specific, logical addresses are assigned based on the topology of the network and can be changed as needed. The most common form of logical network addressing is Internet Protocol (IP) addressing, which includes both IPv4 and IPv6 addresses. IPv4 and IPv6 operate at the network layer (Layer 3) of the OSI model. The function of the Network Layer is to handle the routing of data packets between devices across different networks. This involves logical addressing, packet forwarding, and routing through intermediate routers to reach the final destination. IPv4 Network Addressing An IPv4 address is a 32-bit numeric address that identifies each device on a network. At its core, an IPv4 address is a 32-bit binary number that a network and computer see as a binary sequence of 32 ones and zeros. For example, the following binary sequence is a valid IP address written in binary notation: The representation of an IPv4 address is simplified by breaking the ones and zeros into four equal 8-bit octets, which are separated by a dot: For human readability and to further simplify representation, each of the four octets is converted from binary format to its decimal equivalent: This address is written in a dotted-decimal format, consisting of four octets (8-bit segments) separated by periods. This conversion makes an IP address much easier to remember. Imagine trying to remember 32 ones and zeros for an IP address. Each of the four octets can range from 0 to 255, providing a unique identifier for each device within a network. That means the valid IPv4 address block ranges from 0.0.0.0 to 255.255.255.255. Network Mask A network mask, also known as a netmask, is a 32-bit number that is used in conjunction with an IPv4 address to delineate the network and host portions of the address. An IPv4 address is separated into two main parts: the network ID and the host ID, each of which is determined by the netmask. The network ID identifies the particular network to which the IP address belongs and must be the same on hosts within the specific network. The host ID identifies the specific device (host) within that network and must be unique for each host within the particular network. A netmask is composed of a series of contiguous ones (1s) followed by contiguous zeros (0s) in binary notation. Consider the following masks represented in binary and dotted-decimal notation: Each of the values shown is a valid netmask because the ones are contiguous (adjacent) with each other, and the zeros are contiguous with each other. The following is an example of an invalid mask: This value cannot be a netmask because the binary sequence of ones are not contiguous. There is a zero in the second octet essentially between contiguous ones. This binary sequence represents is a special-use IP address of 255.127.0.0. Classless Inter-Domain Routing (CIDR) Notation Classless Inter-Domain Routing (CIDR) notation provides a concise way to represent an IP address along with its associated network prefix. It is used as a shorthand method of indicating the number of contiguous ones in a netmask. For example, the following masks can be represented using three different notations: dotted-decimal, binary, and CIDR. The three notations for each of the different netmasks all refer to the same mask value. Classful Addressing In the early days of the internet, netmasks were divided up based on classes. Classful netmasks refer to the default masks assigned to each of the original IP address classes (A, B, and C; Class D and E are not typically used for netmask assignments). The following table summarizes the classful IP address classes: Summary of classful IP address classes. These masks were rigid and predetermined, meaning they did not offer flexibility for creating smaller or more specific sub-networks (subnets) within a more extensive network. This inflexibility had several implications, most notably a waste of unused IP addresses and the inability to subdivide a network into smaller networks. Additionally, the classful IP address classes determined the number of networks and the number of hosts per network by the first octet. Classful addressing has, for the most part, been replaced by classless addressing (CIDR). Understanding classful subnet masks provides a foundational understanding of IP networking and a starting point when subnetting a network into smaller subnets. Classless Addressing A subnet mask (netmask) can have values other than 255 in its initial octets. For example, this is a valid subnet mask: Notice the ones are still contiguous even though they don't fill the entire third octet. The address can be converted from binary notation to its dotted-decimal equivalent by converting the third octet into decimal. Binary 11000000 = 192 decimal. This example is referred to as a classless subnet mask. It is classless because it falls outside the previously mentioned classful subnet mask values (/8, /16, and /24). What is the CIDR notation for this classless subnet mask? Count the ones, and you determine the CIDR notation for the 255.255.192.0 mask is /18. Network ID and Host ID How does a network node (host) know what portion of its IP address is the network ID and which is the host ID? We stated earlier that the purpose of the netmask is to determine what portion of an IP address is the network ID and what portion is the host ID. The ones in the netmask determine the network portion of the IP address, and the zeros determine the host portion. For example, given an IP address of 192.168.10.20 and a netmask of 255.255.255.0, notice the IP address is delineated at the point where the ones in the subnet mask end and the zeros begin: When representing the network ID and host ID in dotted-decimal notation, each octet must contain a decimal number. For the example above, the network ID is only three octets in length (192.168.10). Zeros must be appended to fill out the remaining empty octets. The network ID is:192.168.10.0. Similarly, the host ID must contain decimal numbers in all octets. This means we need to prepend zeros to fill out the empty octets. The host ID is:0.0.0.20 Next, consider the same IP address of 192.168.10.20 and a subnet mask of 255.255.0.0. Notice how the change in the subnet mask adjusts the network ID and the host ID. The network ID is:192.168.0.0 The host ID is:0.0.10.20 Thirdly, notice the network ID and host ID with the same given IP address of 192.168.10.20 and a subnet mask of 255.0.0.0. The network ID is:192.0.0.0 The host ID is:0.168.10.20 What relevance does the network ID and host ID have in the real world of networking? Every node in the same network subnet must share the same network ID and a unique host ID if the devices are intended to communicate with each other: A network diagram of a subnet with network ID and host IDs emphasized. In the above example, the blue portion of each node's IP address is the same and indicates the network ID. The green part of each node's IP address is arbitrary but unique for this local subnet. How can we be sure the network ID and host IDs are accurate for this diagram? The network ID and subnet mask are given as 192.168.10.0/24. With a /24 subnet mask, the first three octets of the IP address are the network ID, and the fourth octet is the host ID. If all IP addresses on this subnet are not unique, we have what is called a duplicate IP address (more about this later). Using the given IP subnet of 192.168.10.0/24, how many total hosts can be placed on this network subnet? Remember, all octets range from decimal 0 to decimal 255. These values are based on the fact that each octet is 8 bits in length and represents the range from binary 00000000 (decimal 0) to binary 11111111 (decimal 255). At first, the answer appears to be 256 hosts per subnet using a subnet mask of /24. However, there are reserved addresses in this range: This means the 192.168.10.0/24 subnet can have from 1 to 254 hosts. Public versus Private IPv4 Network Addressing Public IP addresses are globally unique and routable on the public internet, are provisioned by the Internet Assigned Numbers Authority (IANA), and handed out by Regional Internet Registries to organizations and individuals. Public IP addresses facilitate communication between devices over the internet. Private IP addresses are implemented within local networks and are not routable on the public internet. Devices using these addresses can communicate within the local network but require Network Address Translation (NAT) to communicate with external networks. Public IPv4 Addresses Public IP addresses are globally unique identifiers assigned to devices that need to communicate over the public internet. These addresses are routable on the internet and are necessary for any device or service that requires direct access from outside the local network. The Internet Assigned Numbers Authority (IANA) is the organization responsible for the global allocation of IP address space, which is then distributed by Regional Internet Registries to organizations and individuals based on specific criteria and policies. The essential characteristic of public IP addresses is their global uniqueness, ensuring that each address is distinct across the entire internet. This uniqueness allows routers to accurately direct traffic to the correct destination anywhere in the world. Public IP addresses enable the functioning of the internet by facilitating the routing and delivery of data between different networks. For example, a web server hosting a company\'s website might be assigned a public IP address such as 203.0.113.10. This address allows users from around the world to access the website. Similarly, Internet Service Providers (ISPs) assign public IP addresses to customers, allowing their devices to communicate with other devices and services on the internet. Unlike private IP addresses, which are used within local networks and require Network Address Translation (NAT) to communicate externally, public IP addresses do not require NAT for direct internet communication. This direct routability makes them essential for public-facing services and devices, such as websites, email servers, and other online services. Private IPv4 Addresses Private IPv4 addresses are designated for use within local networks and are not routable on the public internet. Devices using these addresses can communicate within the local network but require Network Address Translation (NAT) to communicate with external networks. Private IP addresses help conserve the global IP address space by allowing organizations to use the same internal address ranges without risk of conflicts. This is particularly useful for home networks, corporate intranets, and other internal services that do not need to be accessible from the internet. For instance, a home router might assign IP addresses from the 192.168.1.0/24 range to devices on the home network, such as 192.168.1.1 and 192.168.1.2. Similarly, a company might use the 10.0.0.0/8 range for its internal network, subdividing it into different subnets for various departments like HR and IT. The use of private IP addresses requires NAT when these devices need to communicate with the public internet. NAT converts private IP addresses into a public IP address, enabling multiple devices within a private network to use a single public IP address for external communication. This process not only conserves public IP addresses but also adds a layer of security by masking the internal network structure from external networks. We will cover NAT in detail in a later section. Private IP address ranges as defined by RFC1918. Request for Comments 1918 (RFC1918) RFC 1918 (Request for Comments 1918) is a memorandum published by the Internet Engineering Task Force (IETF) that specifies IP address ranges designated for private use within local networks. These ranges fall within the traditional classful address space but are designated for private, internal network use. They are not routable on the public internet, meaning they cannot be used for direct communication over the internet. Instead, they are intended for use within private networks, such as home networks, corporate intranets, and other internal environments. RFC 1918, titled \"Address Allocation for Private Internets,\" was published and ratified in February 1996. The primary purpose of RFC 1918 is to conserve the global IP address space and allow organizations to use these private addresses internally without the risk of conflicts with public IP addresses. By using private IP addresses, organizations can build large networks without needing a corresponding number of public IP addresses. Each IP address range defined by RFC1918 is associated with a default prefix that indicates the number of bits used for the network portion of the address. This default prefix determines how the address space is divided into network and host portions. Automatic Private Internet Protocol Address (APIPA) Automatic Private IP Addressing (APIPA) is a feature used in Windows-based operating systems that allows a device to automatically assign itself an IP address from a specific range when a DHCP server is unavailable. The APIPA range is 169.254.0.0 to 169.254.255.255, with a default subnet mask of 255.255.0.0. APIPA enables devices on the same local network segment to communicate with each other without requiring manual IP address configuration or a DHCP server. When a device configured to use DHCP cannot contact a DHCP server to obtain an IP address, it will automatically assign itself an IP address from the APIPA range. This ensures that basic network communication can still occur within the local network. Windows assignment of an APIPA address to a NIC. Notice that Windows has "DHCP Enabled: Yes" and has unsuccessfully attempted to obtain an IP address automatically. The basic steps used by a device in configuring an APIPA include: DHCP Request: When a device starts up and it is configured to obtain an IP address automatically, it attempts to obtain an IP address from a DHCP server. DHCP Failure: If no DHCP server responds to the request, the device automatically assigns itself a tentative IP address from the APIPA range. Address Conflict Detection: The device checks to ensure that the chosen APIPA address is not already in use by broadcasting an ARP request. If a conflict is detected, the device selects another address from the APIPA range and repeats the process. If no conflict is detected, the tentative address is assigned. Communication: Once an APIPA address is assigned and verified, the device can communicate with other devices on the same local network segment that also has APIPA addresses. APIPA is beneficial in small networks, such as home or small office networks, where DHCP servers may only sometimes be reliable. For example, if the DHCP server in a small office network goes down, devices on the network can still communicate with each other using APIPA addresses. This ensures minimal disruption to local network services and allows basic file sharing, printing, and other local network activities to continue. APIPA addresses are limited to local communication only, meaning they can only be used within the same local network segment and are not routable to other networks. This restricts devices with APIPA addresses from communicating with devices on different networks or accessing the internet. Additionally, APIPA is intended as a temporary fallback mechanism, providing basic network connectivity until the DHCP server becomes available again or the network issue is resolved. Consequently, it does not replace the need for a functioning DHCP server in more extensive or more complex networks. Finally, since APIPA addresses are not routable, devices assigned these addresses cannot access external networks or the internet, limiting their functionality to local network services only. Loopback/Localhost Address The loopback address is a special IP address used to test network software without transmitting packets over the actual network interfaces. The most commonly used loopback address is 127.0.0.1 in IPv4, but the entire range of 127.0.0.0 to 127.255.255.255 (127.0.0.0/8) is reserved for loopback purposes. This range is also known as \"localhost,\" which refers to the local computer or device itself. The loopback address allows a network device to send and receive data to itself. It is used primarily for testing and diagnostic purposes. By sending data to the loopback address, you can verify that the TCP/IP stack on the device is functioning correctly without needing to send data over the actual network. There are various scenarios where the loopback address is valid: Local Testing: Before deploying an application on a production network, developers can test it locally using 127.0.0.1 to ensure it operates correctly. Troubleshooting: Network administrators can use the loopback address to troubleshoot network stack issues. If a ping to 127.0.0.1 fails, it indicates a problem with the network configuration or hardware on the device itself. Localhost Configuration: Many software applications and services are configured to use the loopback address (localhost) for local communication. For example, a web server running on a local machine can be accessed via http://localhost or http://127.0.0.1. Subnetting IPv4 subnetting is the process of dividing a more extensive IP network into smaller, more manageable sub-networks (subnets). This division is achieved by modifying the subnet mask, which determines how the IP address is split into network and host portions. Subnetting helps optimize network performance, enhance security, and better utilize IP address space. Subnetting is crucial for several reasons. Firstly, it allows for the efficient use of IP address space by creating subnets that match the size needed for specific segments of the network. This means that IP addresses are not wasted and can be allocated more effectively. Secondly, subnetting improves network performance by reducing the size of broadcast domains, which decreases network congestion and enhances overall performance. Thirdly, subnetting enhances security by segmenting the network into smaller subnets, making it easier to apply security policies and isolate network traffic. Finally, subnetting simplifies network management by organizing and managing network resources more effectively, particularly in large networks. By understanding and implementing subnetting, network administrators can optimize network efficiency, performance, and security. Subnetting Examples Subnetting entails taking bits from the host segment of the IP address to generate extra network bits. This process changes the default subnet mask to create a custom subnet mask, which defines the size and structure of the subnets. The following steps are used for subnetting: Step 1: Determine the number of subnets and hosts per subnet needed: Calculate the number of subnets required and the number of hosts per subnet based on network design and requirements. Step 2: Check the feasibility of the given subnet to accommodate the requirements. Step 3: Determine the number of bits required to provide the required number of subnets. Step 4: Borrow bits from the host portion to accommodate the required number of subnets: Adjust the subnet mask to include more network bits to meet the calculated requirement for the number of networks and number of hosts per network. Step 5: Calculate the Subnet Address Ranges: Determine the IP address ranges for each subnet. SUBNETTING EXAMPLE \#1 Let's subnet the following Class C network: Original given network:192.168.1.0/24 Original given subnet mask:255.255.255.0 Requirements:4 subnets 50 hosts per subnet Minimize wasted host addresses With the requirements known, follow the steps for subnetting: The network requirement is four subnets, with at least 50 hosts per subnet. To check the feasibility of subnetting the given network into four subnets, each with at least 50 hosts, we need to ensure the given IP subnet can accommodate the required number of subnets and hosts. This is accomplished by verifying the total number of addresses required for usable and reserved addresses does not exceed 256 (for the given subnet of /24). Each subnet needs 50 usable hosts (addresses). However, we must include two reserved addresses for network ID and broadcast) for a total of: 50 usable + 2 reserved = 52 total addresses per subnet. Total hosts required for 4 subnets = 52 \* 4 = 208 Since 208 is less than 256, the given subnet is feasible and will support four subnets of at least 50 usable hosts per subnet. Determine the number of bits required to provide the desired number of subnets. Number of required subnets = 4 2Number of bits ≥ 4 22 = 4 Two network bits are required to provide four subnets. Borrow two network bits from the host portion to accommodate the required number of subnets: This is accomplished by changing the subnet mask from /24 to /26. Here's what the subnetting process looks like: There is one other value that will make the next step easier which is "block size". The block size refers to the total usable addresses and reserved addresses in a subnet, and is calculated as 2Number of host bits which, in the example above, is 26 = 64. Determine the IP address ranges for each subnet. The previous table illustrates the process for subnetting the Class C 192.168.1.0/24 subnet into four subnets, each with at least 50 hosts per subnet. This example limits the waste of unused IP addresses while providing additional addresses over the required 50 as padding or overhead. It's important to understand that it may not be possible to match the required number of host addresses exactly. The reason is simple binary, as seen here: Using the previous table and assuming our previous Class C subnetting example, notice that our subnet block sizes can range from 1 to 256 (following a pattern of power of 2). The required 50 host addresses fall between block sizes 32 and 64. If we had performed a feasibility calculation using a block size of 32, we would have determined that we would not have had sufficient host addresses. Consequently, we moved up the block size to 64, which passed a feasibility test and met the requirements of 4 subnets with at least 50 hosts per subnet. Any block size over 64 would have worked, except that our requirements state that we must minimize wasted addresses. SUBNETTING EXAMPLE \#2 Let's subnet the following Class B network: Original given network:172.16.0.0/16 Original given subnet mask:255.255.0.0 Requirements:Minimum of 10 subnets Minimum of 2,048 hosts per subnet Minimize wasted host addresses With the requirements known, follow the steps for subnetting: The network requirement is any number of subnets, with at least 2048 hosts per subnet. To check the feasibility of subnetting the given network into any number of subnets, each with at least 2,048 hosts, we need to ensure the given IP subnet can accommodate the required number of subnets and hosts. This is accomplished by verifying the total number of addresses required for usable and reserved addresses does not exceed 216 = 65,536 (for the given subnet of /16). Each subnet needs 2,048 usable hosts (addresses). However, we must include two reserved addresses for network ID and broadcast) for a total of: 2048 usable + 2 reserved = 2,050 total addresses per subnet. Total hosts required for 10 subnets is 2,050 \* 10 = 20,500. Since 20,500 is less than 65,536, the given subnet is feasible and will support ten subnets of at least 2,048 usable hosts per subnet. Determine the number of bits required to provide the desired number of subnets. Number of required subnets = 10 2Number of bits ≥ 10 24 = 16 Four network bits are required to provide at least ten subnets. Borrow network bits from the host portion to accommodate the required number of subnets: This is accomplished by changing the subnet mask from /16 to /20. Here's what the subnetting process looks like: The previous table illustrates the process for subnetting the Class B 172.16.0.0/16 subnet into ten subnets, each with at least 2,048 usable host addresses per subnet. This example limits the waste of unused IP addresses while providing additional addresses over the required 2,048 as padding or overhead. On the surface, this subnetting example is still wasting addresses. After all, we needed 2,048 addresses per subnet and ended up with 4,094. Remember, we must follow powers of 2, and from the table below, you can see that our only choice was to choose four borrowed bits. Again, simple binary proves the point as seen here: Classless Inter-Domain Routing (CIDR) Classless Inter-Domain Routing (CIDR) is a way to allocate IP addresses and route traffic on a network. It was created to replace the older method that divided IP addresses into fixed classes (A, B, C) and allows for more flexible and efficient use of IP addresses. In the older method of classful IP address assignment, the classes were fixed in size according to the /8, /16, and /24 subnets. This meant that any /8 network had up to 16,777,214 available hosts, the /16 network had up to 65,535 available hosts, and the /24 network had up to 254 available hosts. Conversely, CIDR allows for IP address blocks of any size. CIDR also allows the combination of small networks into one larger network. This results in simplier routing and reduced size of routing tables. For example, a CIDR block of 192.168.0.0/22 covers the address range from 192.168.0.0 to 192.168.3.255. CIDR allows for slash (/) notation where a slash (/) is followed with how many bits are used for the network ID portion of the IP address. For example, 192.168.10.0/24 means the first 24 bits identify the network, and the remaining 8 bits identify the host IDs wh\\ithin that network. Variable Length Subnet Mask (VLSM) Variable Length Subnet Mask (VLSM) allows network administrators to divide an IP address space into subnets of different sizes by using subnet masks of varying lengths. This technique optimizes IP address usage by creating subnets that match the specific size requirements of different network segments, thereby reducing wastage. VLSM also enhances network design by providing greater flexibility, making it especially useful in large and complex networks. For example, starting with a network like 192.168.1.0/24, you can create multiple subnets of different sizes: 192.168.1.0/26, which supports 62 devices; 192.168.1.64/27, which supports 30 devices; and 192.168.1.96/28, which supports 14 devices. By using VLSM, network administrators can optimize IP address allocation and improve network efficiency. Modern Network Environments Completion requirements View EXAM OBJECTIVES COVERED IN THIS SECTION 1.8 Summarize evolving use cases for modern network environments. Understanding the evolving use cases and technologies that drive modern network environments is essential in the dynamic networking world. This chapter explores critical advancements such as IPv6 network addressing, Software-Defined Networking (SDN), and Software-Defined Wide Area Networking (SD-WAN). These technologies enhance network scalability, performance, and management by addressing the limitations of traditional networking approaches. Additionally, we delve into security frameworks like Zero Trust Architecture (ZTA) and Secure Access Service Edge (SASE), which ensure robust security in distributed and cloud-based environments. Finally, we examine Infrastructure as Code (IaC), a practice that uses automation and code to manage infrastructure, ensuring consistency and efficiency. These topics provide a comprehensive understanding of the innovations shaping modern network infrastructure. IPv6 Network Addressing IPv6 (Internet Protocol version 6) is designed to address the limitations of its predecessor, IPv4. As the backbone of internet communication, IP enables devices to locate and connect with each other across the globe. The transition to IPv6 is driven by the exponential growth of internet-connected devices, which has led to the exhaustion of the IPv4 address space. IPv6 not only provides a vastly larger address space but also introduces a range of enhancements that improve network functionality, security, and performance. IPv6 uses 128-bit addresses, significantly expanding the number of available IP addresses compared to the 32-bit address space of IPv4. This expansion accommodates the growing number of devices and ensures that there will be sufficient addresses for the foreseeable future. Additionally, IPv6 simplifies network management and configuration by excluding the need for Network Address Translation (NAT), which has been widely used to conserve IPv4 addresses. Comparison of IPv4 and IPv6. Notice the number of IPv6 addresses. The following table compares billions to undecillions. Comparison of IPv4's billions of addresses and IPv6's undecillions of addresses. One of the key challenges in adopting IPv6 is ensuring compatibility with existing IPv4 infrastructure. Various techniques, such as tunneling and dual-stack implementation, facilitate a smooth transition and interoperability between IPv4 and IPv6 networks. These methods enable organizations to migrate to IPv6 gradually while maintaining connectivity with IPv4 systems. Overall, IPv6 addressing is a critical advancement that supports the continued growth and evolution of the internet, offering enhanced security, improved routing efficiency, and greater flexibility in network design. IPv6 and IPv4 Datagrams Datagrams, or packets, in IPv4 and IPv6, serve the same fundamental purpose of transporting data across networks. However, there are several key differences and similarities between IPv4 and IPv6 datagrams in terms of structure, features, and capabilities. The IPv6 datagram consists of a header and payload but with a simplified and fixed header structure compared to IPv4. IPv4 header and datagram. IPv6 header and datagram. IPv6 Address Structure IPv6 addresses consist of 128 bits and serve as identifiers for individual interfaces and groups of interfaces. The following summarizes the address structure and notation of an IPv6 address. IPv6 addresses are often displayed in their compressed form for convenience, but the full, expanded form is used when necessary for clarity, consistency, or technical requirements. Understanding both forms and knowing when to use each is important for effectively working with IPv6 addresses. To simplify notation, enhance readability, and when creating documentation, IPv6 supports leading zero suppression and zero compression. Here are the steps in fully compressing an IPv6 address: There may be times when an IPv6 address requires expansion, such as for consistency, educational purposes, or when entering addresses into configuration files. The steps to expand a compressed IPv6 address are shown here: IPv6 Prefixes and Interface ID IPv6 addresses are composed of a network prefix (Network ID) and an interface identifier (Interface ID). The prefix length can vary depending on the network configuration, ranging from a few bits to the more common 64 bits. The interface ID is then derived from the remaining bits of the 128-bit address. While the typical structure uses a 64-bit prefix and a 64-bit interface ID, the IPv6 protocol supports flexibility in prefix lengths to accommodate various network designs. The network prefix, also known as the network identifier (ID) or network portion, designates a specific subnet or network. The length of the network prefix is variable and is specified using CIDR (Classless Inter-Domain Routing) notation. The CIDR notation indicates the number of bits used for the network prefix. The Interface ID is the portion of the IPv6 address that uniquely identifies an interface on a host within the network. The length of the Interface ID is determined by subtracting the prefix length from 128 bits. IPv6 prefix and interface ID. The following table illustrates examples of variable length prefix and interface IDs with each prefix and corresponding interface ID adding up to 128 bits. Variable IPv6 prefix and interface ID example. IPv6 Unicast and Link-Local Addresses IPv6 unicast addressing includes essential types like global unicast and link-local addresses. Global unicast addresses (GUA) are globally unique and routable on the IPv6 internet, similar to public IPv4 addresses. They typically start with the prefix 2000::/3 and are structured with a 48-bit global routing prefix, a 16-bit subnet ID, and a 64-bit interface ID. This structure allows for hierarchical addressing and efficient routing. Unicast traffic type. Link-local addresses, on the other hand, facilitate communication within a single network segment or link and are not routable beyond the local link. These addresses always start with the prefix fe80::/10 and are automatically assigned to all IPv6-enabled interfaces. Link-local addresses play a crucial role in network operations, such as neighbor discovery and address autoconfiguration. IPv6 Multicast and Anycast Addresses IPv6 eliminates the concept of broadcast addresses, which were used in IPv4 for sending traffic to all nodes on a network. Instead, IPv6 employs multicast and anycast addressing to efficiently handle similar tasks. Multicast addresses are used to forward a single packet to multiple destinations simultaneously, reducing the need for multiple unicast transmissions and minimizing network load. These addresses always start with the prefix ff00::/8. Multicast traffic type. Anycast addresses are assigned to multiple interfaces, typically on different nodes. A packet addressed to an anycast address is routed to the nearest interface with that address, according to routing distance. This method is efficient for load balancing and redundancy. Anycast traffic type. Mitigating Address Exhaustion One of the primary motivations behind the development of IPv6 was to address the issue of IPv4 address exhaustion. The rapid expansion of internet-connected devices has outstripped the capacity of IPv4's 32-bit address space, which supports approximately 4.3 billion unique addresses. IPv6, with its 128-bit address space, provides a vast number of addresses, ensuring sufficient availability for the foreseeable future. IPv6 addresses the limitations of IPv4 by providing a vastly larger address space and incorporating features that enhance address allocation and management. The 128-bit addressing scheme, hierarchical structure, and support for multiple address types ensure that IPv6 can handle the growing number of internet-connected devices. By eliminating the need for NAT, IPv6 also simplifies network design and improves connectivity. These innovations collectively mitigate the issue of address exhaustion, ensuring the continued growth and scalability of the internet. IPv6 Compatibility Requirements The transition from IPv4 to IPv6 requires compatibility mechanisms because IPv6 and IPv4 are not inherently interoperable. Devices using IPv6 cannot communicate directly with devices using IPv4, or vice versa, without assistance. To ensure seamless communication during this transition period, network administrators employ techniques such as tunneling, dual stack, and NAT64. Dual sta