itp-unit-3.docx
Document Details
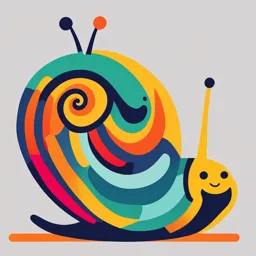
Uploaded by SuaveLitotes
Tags
Related
- Sensation and Perception Year 11 Psychology PDF
- Psychology 40S Chapter 3 Test - Sensation and Perception PDF
- PSGY1010 Cognitive Psychology 1 Perception 1 PDF
- PSY 1000 Introduction to Psychology Chapter 5 Study Questions - PDF
- Sensation and Perception PDF - Psychology Notes
- PSY 101: Introductory Psychology - Sensation & Perception - Michigan State University PDF
Full Transcript
Why study sensation and perception? Without sensations to tell us what is outside our own mental world, we would live entirely in our own minds, separate from one another and unable to find food or any other basics that sustain life. Sensations are the mind's window to the world that exists arou...
Why study sensation and perception? Without sensations to tell us what is outside our own mental world, we would live entirely in our own minds, separate from one another and unable to find food or any other basics that sustain life. Sensations are the mind's window to the world that exists around us. Without perception, we would be unable to understand what all those sensations mean---perception is the process of interpreting the sensations we experience so that we can act upon them. Information about the world has to have a way to get into the brain, where it can be used to determine actions and responses. The way into the brain is through the sensory organs and the process of sensation. **WHAT IS SENSATION?** **3.1** How does sensation travel through the central nervous system, and why are some sensations ignored? **Sensation** occurs when special receptors in the sense organs---the eyes, ears, nose, skin, and taste buds---are activated, allowing various forms of outside stimuli to become neural signals in the brain. (This process of converting outside stimuli, such as light, into neural activity is called **transduction**.) Let's take a closer look at these special receptors. **SENSORY RECEPTORS** The *sensory receptors* are specialized forms of neurons, the cells that make up the nervous system. Instead of receiving neurotransmitters from other cells, these receptor cells are stimulated by different kinds of energy---for example, the receptors in the eyes are stimulated by light, whereas the receptors in the ears are activated by vibrations. Touch receptors are stimulated by pressure or temperature, and the receptors for taste and smell are triggered by chemical substances. **SENSORY THRESHOLDS** Ernst Weber (1795--1878) did studies trying to determine the smallest difference between two weights that could be detected. His research led to the formulation known as Weber's law of **just noticeable differences (jnd,** or the **difference threshold**). A jnd is the smallest difference between two stimuli that is detectable 50 percent of the time, and Weber's law simply means that whatever the difference between stimuli might be, it is always a *constant*. If to notice a difference the amount of sugar a person would need to add to a cup of coffee that is already sweetened with 5 teaspoons is 1 teaspoon, then the percentage of change needed to detect a just noticeable difference is one-fifth, or 20 percent. So if the coffee has 10 teaspoons of sugar in it, the person would have to add another 20 percent, or 2 teaspoons, to be able to taste the difference half of the time. Most people would not typically drink a cup of coffee with 10 teaspoons of sugar in it, let alone 12 teaspoons, but you get the point. Gustav Fechner (1801--1887) expanded on Weber's work by studying something he called the **absolute threshold** (Fechner, 1860). An absolute threshold is the lowest level of stimulation that a person can consciously detect 50 percent of the time the stimulation is present. (Remember, the jnd is detecting a difference *between two* stimuli.) For example, assuming a very quiet room and normal hearing, how far away can someone sit and you might still hear the tick of their analog watch on half of the trials? For some examples of absolute thresholds for various senses, see Table 3.1. I've heard about people being influenced by stuff in movies and on television, things that are just below the level of conscious awareness. Is that true? Stimuli that are below the level of conscious awareness are called *subliminal stimuli*. (The word *limin* means "threshold," so *sublimin* means "below the threshold.") These stimuli are just strong enough to activate the sensory receptors but not strong enough for people to be consciously aware of them. Many people believe that these stimuli act upon the unconscious mind, influencing behavior in a process called *subliminal perception*. At one time, many people believed that a market researcher named James Vicary had demonstrated the power of subliminal perception in advertising. It was five years before Vicary finally admitted that he had never conducted a real study (Merikle, 2000; Pratkanis, 1992). Furthermore, many researchers have gathered scientific evidence that subliminal perception does not work in advertising (Bargh et al., 1996; Broyles, 2006; Moore, 1988; Pratkanis & Greenwald, 1988; Trappey, 1996; Vokey & Read, 1985). This is not to say that subliminal perception does not exist---there is a growing body of evidence that we process some stimuli without conscious awareness, especially stimuli that are fearful or threatening (LeDoux & Phelps, 2008; Öhman, 2008). In this effort, researchers have used *event-related potentials* (ERPs) and functional magnetic resonance imaging (fMRI) to verify the existence of subliminal perception and associated learning in the laboratory (Babiloni et al., 2010; Bernat et al., 2001; Fazel-Rezai & Peters, 2005; Sabatini et al., 2009). However, as in about every other case where subliminal perception has reportedly occurred, these studies use stimuli that are *supraliminal---*"above the threshold"---and detectable by our sensory systems. However, they are below the level of conscious perception and participants are not aware or conscious that they have been exposed to the stimuli due to masking or manipulation of attention. Furthermore, the stimuli typically influence automatic reactions (such as an increase in facial tension) rather than direct voluntary behaviors (such as going to buy something suggested by advertising). The real world is full of complex motives that are not as easily influenced as one might think (Pratkanis, 1992). Even the so-called hidden pictures that some artists airbrush into the art in advertisements aren't truly subliminal---if someone points one out, it can be seen easily enough. **HABITUATION AND SENSORY ADAPTATION** In Chapter Two it was stated that the lower centers of the brain filter sensory stimulation and "ignore" or prevent conscious attention to stimuli that do not change. The brain is only interested in changes in information. That's why people don't really "hear" the noise of the air conditioner unless it suddenly cuts off or the noise made in some classrooms unless it gets very quiet. Although they actually are *hearing* it, they aren't paying attention to it. This is called **habituation**, and it is the way the brain deals with unchanging information from the environment. to Chapter Two: The Biological Perspective, p. 70*.* Sometimes I can smell the odor of the garbage can in the kitchen when I first come home, but after a while the smell seems to go away---is this also habituation? Although different from habituation, **sensory adaptation** is another process by which constant, unchanging information from the sensory receptors is effectively ignored. In habituation, the sensory receptors are still responding to stimulation but the lower centers of the brain are not sending the signals from those receptors to the cortex. The process of sensory adaptation differs because the receptor cells *themselves* become less responsive to an unchanging stimulus---garbage odors included---and the receptors no longer send signals to the brain. For example, when you eat, the food that you put in your mouth tastes strong at first, but as you keep eating the same thing, the taste does fade somewhat, doesn't it? Smell, taste, and touch are all subject to sensory adaptation. You might think, then, that if you stare at something long enough, it would also disappear, but the eyes are a little different. Even though the sensory receptors in the back of the eyes adapt to and become less responsive to a constant visual stimulus, under ordinary circumstances the eyes are never entirely still. There's a constant movement of the eyes, tiny little vibrations called "microsaccades" or "saccadic movements" that people don't consciously notice. These movements keep the eyes from adapting to what they see. (That's a good thing, because otherwise many students would no doubt go blind from staring off into space.) **The Science of Seeing** **I've heard that light is waves, but I've also heard that light is made of particles---which is it?** **Light is a complicated phenomenon. Although scientists have long argued over** **the nature of light, they finally have agreed that light has the properties of both waves** **and particles. The following section gives a brief history of how scientists have tried to** **"shed light" on the mystery of light.** **PERCEPTUAL PROPERTIES OF LIGHT: CATCHING THE WAVES** **3.2 What is light, and how does it travel through the various parts of the eye?** **It was Albert Einstein who first proposed that light is actually tiny "packets" of waves.** **These "wave packets" are called *photons* and have specific wavelengths associated with** **them (Lehnert, 2007; van der Merwe & Garuccio, 1994).** **When people experience the physical properties of light, they** **are not really aware of its dual, wavelike and particle-like,** **nature. With regard to its psychological properties, there are three aspects to our perception of light: *brightness, color*, and *saturation*.** ***Brightness* is determined by the amplitude of the wave---how high or how low the wave actually is. The higher the wave, the brighter the light appears to be. Low waves are dimmer. *Color*, or hue, is largely determined by the length of the wave. Long wavelengths (measured in nanometers) are found at the red end of the *visible spectrum* (the portion of the whole spectrum of light that is visible to the** **human eye; see Figure 3.1), whereas shorter wavelengths** **are found at the blue end. (Note that when combining different colors, light behaves differently than pigments or paint. We will look at this distinction when we** **examine perception of color)** The next visual layer is a clear, watery fluid called the *aqueous humor*. This fluid is continually replenished and supplies nourishment to the eye. The light from the visual image then enters the interior of the eye through a hole, called the *pupil*, in a round muscle called the *iris* (the colored part of the eye). The iris can change the size of the pupil, letting more or less light into the eye. That also helps focus the image; people try to do the same thing by squinting. Behind the iris, suspended by muscles, is another clear structure called the *lens*. The flexible lens finishes the focusing process begun by the cornea. In a process called **visual accommodation**, the lens changes its shape from thick to thin, enabling it to focus on objects that are close or far away. The variation in thickness allows the lens to project a sharp image on the retina. People lose this ability as the lens hardens through aging (a disorder called *presbyopia*). Although people try to compensate\* for their inability to focus on things that are close to them, eventually they usually need bifocals because their arms just aren't long enough anymore. Once past the lens, light passes through a large, open space filled with a clear, jelly-like fluid called the *vitreous humor*. This fluid, like the aqueous humor, also nourishes the eye and gives it shape. **RETINA, RODS, AND CONES** The final stop for light within the eye is the *retina*, a lightsensitive area at the back of the eye containing three layers: ganglion cells, bipolar cells, and the **rods** and **cones**, special cells (*photoreceptors*) that respond to the various light waves. (See Figures 3.3a and b.) The rods and the cones are the business end of the retina---the part that actually receives the photons of light and turns them into neural signals to the brain, sending them first to the *bipolar cells* (a type of interneuron; called bipolar or "two-ended" because they have a single dendrite at one end and a single axon on the other; to Chapter Two: The Biological Perspective, p. 57) and then to the retinal *ganglion cells* whose axons form the optic nerve. (See Figure 3.3a.) Figure 3.3 **The Parts of the Retina** \(a) Light passes through ganglion and bipolar cells until it reaches and stimulates the rods and cones. Nerve impulses from the rods and cones travel along a nerve pathway to the brain. (b) On the right of the figure is a photomicrograph of the long, thin rods and the shorter, thicker cones; the rods outnumber the cones by a ratio of about 20 to 1. (c) The blind spot demonstration. Hold the book in front of you. Close your right eye and stare at the picture of the dog with your left eye. Slowly bring the book closer to your face. The picture of the cat will disappear at some point because the light from the picture of the cat is falling on your blind spot **THE BLIND SPOT** The eyes don't adapt to constant stimuli under normal circumstances because of saccadic movements. But if people stare with one eye at one spot long enough, objects that slowly cross their visual field may at one point disappear briefly because there is a "hole" in the retina---the place where all the axons of those ganglion cells leave the retina to become the optic nerve. There are no rods or cones here, so this is referred to as the **blind spot**. You can demonstrate the blind spot for yourself by following the directions in Figure 3.3c. **HOW THE EYE WORKS** **3.3** How do the eyes see, and how do the eyes see different colors? **THROUGH THE EYES TO THE BRAIN** You may want to first look at Figure 3.4 for a moment before reading this section. Light entering the eyes can be separated into the left and right visual fields. Light from the right visual field falls on the left side of each eye's retina; light from the left visual field falls on the right side of each retina. Light travels in a straight line through the cornea and lens; resulting in the image projected on the retina actually being upside down and reversed from left to right as compared to the visual fields. Thank goodness our brains can compensate for this! The areas of the retina can be divided into halves, with the halves toward the temples of the head referred to as the temporal retinas and the halves toward the center, or nose, called the nasal retinas. Look at Figure 3.4 again. Notice that the information from the left visual field (falling on the right side of each retina) goes directly to the right visual cortex, while the information from the right visual field (falling on the left side of each retina) goes directly to the left visual cortex.This is because the axons from the temporal halves of each retina project to the visual cortex on the same side of the brain while the axons from the nasal halves cross over to the visual cortex on the opposite side of the brain. The optic chiasm is the point of crossover. Let's go back now to the photoreceptors in the retina, the rods and cones responsible for different aspects of vision. The rods (about 120 million of them in each eye) are found all over the retina except in the very center, which contains only cones. Rods are sensitive to changes in brightness but not to changes in wavelength, so they see only in black and white and shades of gray. They can be very sensitive because many rods are connected to a single bipolar cell, so that if even only one rod is stimulated by a photon of light, the brain perceives the whole area of those rods as stimulated (because the brain is receiving the message from the single bipolar cell). But because the brain doesn't know exactly what part of the area (which rod) is actually sending the message, the visual acuity (sharpness) is quite low. That's why things seen in low levels of light, such as twilight or a dimly lit room, are fuzzy and grayish. Because rods are located on the periphery of the retina, they are also responsible for peripheral vision. Because rods work well in low levels of light, they are also the cells that allow the eyes to adapt to low light. **Dark adaptation** occurs as the eye recovers its ability to see when going from a brightly lit state to a dark state. (The light-sensitive pigments that allow us to see are able to regenerate or "recharge" in the dark.) The brighter the light was, the longer it takes the rods to adapt to the new lower levels of light (Bartlett, 1965). This is why the bright headlights of an oncoming car can leave a person less able to see for a while after that car has passed. Fortunately, this is usually a temporary condition because the bright light was on so briefly and the rods readapt to the dark night relatively quickly. Full dark adaptation, which occurs when going from more constant light to darkness such as turning out one's bedroom lights, takes about 30 minutes. As people get older this process takes longer, causing many older persons to be less able to see at night and in darkened rooms (Klaver et al., 1998). This age-related change can cause *night blindness*, in which a person has difficulty seeing well enough to drive at night or get around in a darkened room or house. Some research indicates that taking supplements such as vitamin A can reverse or relieve this symptom in some cases (Jacobsen et al., 1995). When going from a darkened room to one that is brightly lit, the opposite process occurs. The cones have to adapt to the increased level of light, and they accomplish this **light adaptation** much more quickly than the rods adapt to darkness---it takes a few seconds at most (Hood, 1998). There are 6 million cones in each eye; of these, 50,000 have a private line to the optic nerve (one bipolar cell for each cone). This means that the cones are the receptors for visual acuity. Cones are located all over the retina but are more concentrated at its very center where there are no rods (the area called the *fovea*). Cones also need a lot more light to function than the rods do, so cones work best in bright light, which is also when people see things most clearly. Cones are also sensitive to different wavelengths of light, so they are responsible for color vision. **PERCEPTION OF COLOR** Earlier you said the cones are used in color vision. There are so many colors in the world ---are there cones that detect each color? Or do all cones detect all colors? Although experts in the visual system have been studying color and its nature for many years, at this point in time there is an ongoing theoretical discussion about the role the cones play in the sensation of color. **THEORIES OF COLOR VISION** Two theories about how people see colors were originally proposed in the 1800s. The first is called the **trichromatic** ("three colors") **theory**. First proposed by Thomas Young in 1802 and later modified by Hermann von Helmholtz in 1852, this theory proposed three types of cones: red cones, blue cones, and green cones, one for each of the three primary colors of light. Most people probably think that the primary colors are red, yellow, and blue, but these are the primary colors when talking about *painting*---not when talking about *light*. Paints *reflect* light, and the way reflected light mixes is different from the way direct light mixes. For example, if an artist were to blend red, yellow, and blue paints together, the result would be a mess---a black mess. The mixing of paint (reflected light) is subtractive, removing more light as you mix in more colors. As all of the colors are mixed, the more light waves are absorbed and we see black. But if the artist were to blend a red, green, and blue light together by focusing lights of those three colors on one common spot, the result would be white, not black. The mixing of direct light is additive, resulting in lighter colors, more light, and when mixing red, blue, and green, we see white, the reflection of the entire visual spectrum. In the trichromatic theory, different shades of colors correspond to different amounts of light received by each of these three types of cones.These cones then fire their message to the brain's vision centers. It is the combination of cones and the rate at which they are firing that determine the color that will be seen. For example, if the red and green cones are firing in response to a stimulus at fast enough rates, the color the person sees is yellow. If the red and blue cones are firing fast enough, the result is magenta. If the blue and green cones are firing fast enough, a kind of cyan color (blue-green) appears Brown and Wald (1964) identified three types of cones in the retina, each sensitive to a range of wavelengths, measured in nanometers (nm), and a peak sensitivity that roughly corresponds to three different colors (although hues/colors can vary depending on brightness and saturation). The peak wavelength of light the cones seem to be most sensitive to turns out to be just a little different from Young and von Helmholtz's original three corresponding colors: Short wavelength cones detect what we see as blue-violet (about 420 nm), medium wavelength cones detect what we see as green (about 530 nm), and long wavelength cones detect what we see as green-yellow (about 560 nm). Interestingly, none of the cones identified by Brown and Wald have a peak sensitivity to light where most of us see red (around 630 nm). Keep in mind though, each cone responds to light across a range of wavelengths, not just its wavelength of peak sensitivity. Depending on the intensity of the light, both the medium and long wavelength cones respond to light that appears red. **THE AFTERIMAGE** The trichromatic theory would, at first glance, seem to be more than adequate to explain how people perceive color. But there's an interesting phenomenon that this theory cannot explain. If a person stares at a picture of the American flag for a little while---say, a minute---and then looks away to a blank white wall or sheet of paper, that person will see an afterimage of the flag. **Afterimages** occur when a visual sensation persists for a brief time even after the original stimulus is removed. The person would also notice rather quickly that the colors of the flag in the afterimage are all wrong---green for red, black for white, and yellow for blue. If you follow the directions for Figure 3.5, in which the flag is yellow, green, and black, you should see a flag with the usual red, white, and blue. Hey, now the afterimage of the flag has normal colors! Why does this happen? The phenomenon of the color afterimage is explained by the second theory of color perception, called the **opponent-process theory** (De Valois & De Valois, 1993; Hurvich & Jameson, 1957), based on an idea first suggested by Edwald Hering in 1874 (Finger, 1994). In opponent-process theory, there are four primary colors: red, green, blue, and yellow. The colors are arranged in pairs, red with green and blue with yellow. If one member of a pair is strongly stimulated, the other member is inhibited and cannot be working---so there are no reddish-greens or bluish-yellows. So how can this kind of pairing cause a color afterimage? From the level of the bipolar and ganglion cells in the retina, all the way through the thalamus, and on to the visual cortical areas in the brain, some neurons (or groups of neurons) are stimulated by light from one part of the visual spectrum and inhibited by light from a different part of the spectrum. For example, let's say we have a red-green ganglion cell in the retina whose baseline activity is rather weak when we expose it to white light. However, the cell's activity is increased by red light, so we experience the color red. If we stimulate the cell with red light for a long enough period of time, the cell becomes fatigued. If we then swap out the red light with white light, the now-tired cell responds even less than the original baseline. Now we experience the color green, because green is associated with a decrease in the responsiveness of this cell. So which theory is the right one? Both theories play a part in color vision. Trichromatic theory can explain what is happening with the raw stimuli, the actual detection of various wavelengths of light. Opponent-process theory can explain afterimages and other aspects of visual perception that occur after the initial detection of light from our environment. In addition to the retinal bipolar and ganglion cells, opponent-process cells are contained inside the thalamus in an area called the lateral geniculate nucleus (LGN). The LGN is part of the pathway that visual information takes to the occipital lobe. It is when the cones in the retina send signals through the retinal bipolar and ganglion cells that we see the red versus green pairings and blue versus yellow pairings. Together with the retinal cells, the cells in the LGN appear to be the ones responsible for opponent-processing of color vision and the afterimage effect. So which theory accounts for color blindness? I've heard that there are two kinds of color blindness, when you can't tell red from green and when you can't tell blue from yellow. **COLOR BLINDNESS** From the mention of red-green and yellow-blue color blindness, one might think that the opponent-process theory explains this problem. But in reality "color blindness" is caused by defective cones in the retina of the eye and as a more general term, *color-deficient vision* is more accurate, as most people with "color blindness" have two type of cones working and can see many colors. There are really three kinds of color-deficient vision. In a very rare type, *monochrome color blindness*, people either have no cones or have cones that are not working at all. Essentially, if they have cones, they only have one type and, therefore, everything looks the same to the brain---shades of gray. The other types of color-deficient vision, or *dichromatic vision*, are caused by the same kind of problem---having one cone that does not work properly. *Protanopia* (red-green color deficiency) is due to the lack of functioning red cones and *deuteranopia* (another type of red-green color deficiency) results from the lack of functioning green cones. In both of these, the individual confuses reds and greens, seeing the world primarily in blues, yellows, and shades of gray. A lack of functioning blue cones is much less common and called *tritanopia* (blue-yellow color deficiency). These individuals see the world primarily in reds, greens, and shades of gray. To get an idea of what a test for color-deficient vision is like, look at Figure 3.6. Why are most of the people with color-deficient vision men? Color-deficient vision involving one set of cones is inherited in a pattern known as *sex-linked inheritance*. The gene for color-deficient vision is *recessive*. To inherit a recessive trait, you normally need two of the genes, one from each parent. to Chapter Eight: Development Across the Life Span, p. 301*.* But the gene for color-deficient vision is attached to a particular chromosome (a package of genes) that helps to determine the sex of a person. Men have one X chromosome and one smaller Y chromosome (named for their shapes), whereas women have two X chromosomes. The smaller Y has fewer genes than the larger X, and one of the genes missing is the one that would suppress the gene for color-deficient vision. For a woman to have color-deficient vision, she must inherit two recessive genes, one from each parent, but a man only needs to inherit *one* recessive gene--- the one passed on to him on his mother's X chromosome. His odds are greater; therefore, more males than females have color-deficient vision. **he Hearing Sense: Can You Hear Me Now?** If light works like waves, then do sound waves have similar properties? The properties of sound are indeed similar to those of light, as both senses rely on waves. But the similarity ends there, as the physical properties of sound are different from those of light. **PERCEPTION OF SOUND: GOOD VIBRATIONS** **3.4** What is sound, and how does it travel through the various parts of the ear? Sound waves do not come in little packets the way light comes in photons. Sound waves are simply the vibrations of the molecules of air that surround us. Sound waves do have the same properties of light waves though---wavelength, amplitude, and *purity*. Wavelengths are interpreted by the brain as the frequency or *pitch* (high, medium, or low). Amplitude is interpreted as *volume*, how soft or loud a sound is. (See Figure 3.7.) Finally, what would correspond to saturation or purity in light is called *timbre* in sound, a richness in the tone of the sound. And just as people rarely see pure colors in the world around us, they also seldom hear pure sounds. The everyday noises that surround people do not allow them to hear many pure tones. Just as a person's vision is limited by the visible spectrum of light, a person is also limited in the range of frequencies he or she can hear. Frequency is measured in cycles (waves) per second, or **hertz (Hz)**. Human limits are between 20 and 20,000 Hz, with the most sensitivity from about 2000 to 4000 Hz, very important for conversational speech. (In comparison, dogs can hear between 50 and 60,000 Hz, and dolphins can hear up to 200,000 Hz.) To hear the higher and lower frequencies of a piece of music on a CD, for example, a person would need to increase the amplitude or volume---which explains why some people like to "crank it up." **THE STRUCTURE OF THE EAR: FOLLOW THE VIBES** The ear is a series of structures, each of which plays a part in the sense of hearing, as shown in Figure 3.8. **THE OUTER EAR** The **pinna** is the visible, external part of the ear that serves as a kind of concentrator, funneling\* the sound waves from the outside into the structure of the ear.The pinna is also the entrance to the **auditory canal** (or ear canal), the short tunnel that runs down to the *tympanic membrane*, or eardrum. When sound waves hit the eardrum, they cause three tiny bones in the middle ear to vibrate. **THE MIDDLE EAR: HAMMER, ANVIL, AND STIRRUP** The three tiny bones in the middle ear are known as the hammer (*malleus*), anvil (*incus*), and stirrup (*stapes*), each name stemming from the shape of the respective bone. The vibration of these three bones amplifies the vibrations from the eardrum. The stirrup, the last bone in the chain, causes a membrane covering the opening of the inner ear to vibrate. **THE INNER EAR** This membrane is called the *oval window*, and its vibrations set off another chain reaction within the inner ear. **Cochlea** The inner ear is a snail-shaped structure called the **cochlea**, which is filled with fluid. When the oval window vibrates, it causes the fluid in the cochlea to vibrate. This fluid surrounds a membrane running through the middle of the cochlea called the *basilar membrane*. **Basilar Membrane and the Organ of Corti** The *basilar membrane* is the resting place of the *organ of Corti*, which contains the receptor cells for the sense of hearing. When the basilar membrane vibrates, it vibrates the organ of Corti, causing it to brush against a membrane above it. On the organ of Corti are special cells called *hair cells*, which are the receptors for sound. When these auditory receptors or hair cells are bent up against the other membrane, it causes them to send a neural message through the **auditory nerve** (which contains the axons of all the receptor neurons) and into the brain, where the auditory cortex will interpret the sounds (the transformation of the vibrations of sound into neural messages is transduction). The louder the sound in the outside world, the stronger the vibrations that stimulate more of those hair cells---which the brain interprets as loudness. I think I have it straight---but all of that just explains how soft and loud sounds get to the brain from the outside. How do we hear different kinds of sounds, like high pitches and low pitches? **PERCEIVING PITCH** **Pitch** refers to how high or low a sound is. For example, the bass tones in the music pounding through the wall of your apartment from the neighbors next door is a low pitch, whereas the scream of a 2-year-old child is a very high pitch. *Very* high. There are three primary theories about how the brain receives information about pitch. The oldest of the three theories, **place theory**, is based on an idea proposed in 1863 by Hermann von Helmholtz and elaborated on and modified by Georg von Békésy, beginning with experiments first published in 1928 (Békésy, 1960). In this theory, the pitch a person hears depends on where the hair cells that are stimulated are located on the organ of Corti. For example, if the person is hearing a high-pitched sound, all of the hair cells near the oval window will be stimulated, but if the sound is low pitched, all of the hair cells that are stimulated will be located farther away on the organ of Corti. **Frequency theory**, developed by Ernest Rutherford in 1886, states that pitch is related to how fast the basilar membrane vibrates. The faster this membrane vibrates, the higher the pitch; the slower it vibrates, the lower the pitch. (In this theory, all of the auditory neurons would be firing at the same time.) So which of these first two theories is right? It turns out that both are right---up to a point. For place-theory research to be accurate, the basilar membrane has to vibrate unevenly---which it does when the frequency of the sound is *above* 1000 Hz. For the frequency theory to be correct, the neurons associated with the hair cells would have to fire as fast as the basilar membrane vibrates. This only works up to 1000 Hz, because neurons don't appear to fire at exactly the same time and rate when frequencies are faster than 1000 times per second. The frequency theory works for low pitches, and place theory works for moderate to high pitches. Is there another explanation? Yes, and it is a third theory, developed by Ernest Wever and Charles Bray, called the **volley principle** (Wever, 1949; Wever & Bray, 1930), which appears to account for pitches from about 400 Hz up to about 4000. In this explanation, groups of auditory neurons take turns firing in a process called *volleying*. If a person hears a tone of about 3000 Hz, it means that three groups of neurons have taken turns sending the message to the brain---the first group for the first 1000 Hz, the second group for the next 1000 Hz, and so on. **TYPES OF HEARING IMPAIRMENTS** *Hearing impairment* is the term used to refer to difficulties in hearing. A person can be partially hearing impaired or totally hearing impaired, and the treatment for hearing loss will vary according to the reason for the impairment. **3.5** Why are some people unable to hear, and how can their hearing be improved? **CONDUCTION HEARING IMPAIRMENT** *Conduction hearing impairment* means that sound vibrations cannot be passed from the eardrum to the cochlea.The cause might be a damaged eardrum or damage to the bones of the middle ear (usually from an infection). In this kind of impairment, hearing aids may be of some use in restoring hearing. **NERVE HEARING IMPAIRMENT** In *nerve hearing impairment*, the problem lies either in the inner ear or in the auditory pathways and cortical areas of the brain. Normal aging causes loss of hair cells in the cochlea, and exposure to loud noises can damage hair cells. *Tinnitus* is a fancy word for an extremely annoying ringing in one's ears, and it can also be caused by infections or loud noises---including loud music in headphones, so you might want to turn down that music player! Because the damage is to the nerves or the brain, nerve hearing impairment cannot be helped with ordinary hearing aids, which are basically sound amplifiers. A technique for restoring some hearing to those with nerve hearing impairment makes use of an electronic device called a *cochlear* *implant*. This device sends signals from a microphone worn behind the ear to a sound processor worn on the belt or in a pocket, which then translates those signals into electrical stimuli that are sent to a series of electrodes implanted directly into the cochlea, allowing transduction to take place and stimulating the auditory nerve. (See Figure 3.9.) The brain then processes the electrode information as sound. **Chemical Senses: It Tastes Good** **and Smells Even Better** **3.6** How do the senses of taste and smell work, and how are they alike? The sense of taste (taste in food, not taste in clothing or friends) and the sense of smell are very closely related. Have you ever noticed that when your nose is all stopped up, your sense of taste is affected, too? That's because the sense of taste is really a combination of taste and smell. Without the input from the nose, there are actually only four, and possibly five, kinds of taste sensors in the mouth. **GUSTATION: HOW WE TASTE THE WORLD** **TASTE BUDS** *Taste buds* are the common name for the taste receptor cells, special kinds of neurons found in the mouth that are responsible for the sense of taste, or **gustation**. Most taste buds are located on the tongue, but there are a few on the roof of the mouth, the cheeks, and under the tongue as well. How sensitive people are to various tastes depends on how many taste buds they have; some people have only around 500, whereas others have 20 times that number. The latter are called "supertasters" and need far less seasoning in their food than those with fewer taste buds (Bartoshuk, 1993). So taste buds are those little bumps I can see when I look closely at my tongue? No, those "bumps" are called *papillae*, and the taste buds line the walls of these papillae. (See Figure 3.10.) Each taste bud has about 20 receptors that are very similar to the receptor sites on receiving neurons at the synapse. to Chapter Two: The Biological Perspective, p. 52*.* In fact, the receptors on taste buds work exactly like receptor sites on neurons---they receive molecules of various substances that fit into the receptor like a key into a lock. Taste is often called a chemical sense because it works with the molecules of foods people eat in the same way the neural receptors work with neurotransmitters. When the molecules (dissolved in saliva) fit into the receptors, a signal is fired to the brain, which then interprets the taste sensation. What happens to the taste buds when I burn my tongue? Do they repair themselves? I know when I have burned my tongue, I can't taste much for a while, but the taste comes back. In general, the taste receptors get such a workout that they have to be replaced every 10 to 14 days (McLaughlin & Margolskee, 1994). And when the tongue is burned, the damaged cells no longer work. As time goes on, those cells get replaced and the taste sense comes back. **THE FIVE BASIC TASTES** In 1916 a German psychologist named Hans Henning proposed that there are four primary tastes: sweet, sour, salty, and bitter. Lindemann (1996) supported the idea that there is a fifth kind of taste receptor that detects a pleasant "brothy" taste associated with foods like chicken soup, tuna, kelp, cheese, and soy products, among others. Lindemann proposed that this fifth taste be called *umami*, a Japanese word first coined in 1908 by Dr. Kikunae Ikeda of Tokyo Imperial University to describe the taste. Dr. Ikeda had succeeded in isolating the substance in kelp that generated the sensation of umami---glutamate (Beyreuther et al., 2007). to Chapter Two: The Biological Perspective, p\. 53*.* Glutamate exists not only in the foods listed earlier, but is also present in human breast milk and is the reason that the seasoning MSG---monosodium *glutamate*---adds a pleasant flavor to foods. The five taste sensations work together, along with the sense of smell and the texture, temperature, and "heat" of foods, to produce thousands of taste sensations. Although researchers used to believe that certain tastes were located on certain places on the tongue, it is now known that all of the taste sensations are processed all over the tongue (Bartoshuk, 1993). Just as individuals and groups can vary on their food preferences, they can also vary on level of perceived sweetness. For example, obese individuals have been found to experience less sweetness than individuals who are not obese; foods that are both sweet and high in fat tend to be especially attractive to individuals who are obese (Bartoshuk et al., 2006). Such differences (as well as genetic variations like the supertasters) complicate direct comparison of food preferences. One possible solution is to have individuals rate taste in terms of an unrelated "standard" sensory experience of known intensity, such as the brightness of a light or loudness of a sound or preference in terms of all pleasurable experiences, and not just taste (Bartoshuk et al., 2005; Snyder & Bartoshuk, 2009). Turning our attention back to how things taste for us as individuals, have you ever noticed that when you have a cold, food tastes very bland? Everything becomes bland or muted because you can taste only sweet, salty, bitter, sour, and umami---and because your nose is stuffed up with a cold, you don't get all the enhanced variations of those tastes that come from the sense of smell. **THE SENSE OF SCENTS: OLFACTION** Like the sense of taste, the sense of smell is a chemical sense. The ability to smell odors is called **olfaction,** or the **olfactory sense**. The outer part of the nose serves the same purpose for odors that the pinna and ear canal serve for sounds: Both are merely ways to collect the sensory information and get it to the part of the body that will translate it into neural signals. The part of the olfactory system that transduces odors---turns odors into signals the brain can understand---is located at the top of the nasal passages. This area of olfactory receptor cells is only about an inch square in each cavity yet contains about 10 million olfactory receptors. (See Figure 3.11.) **OLFACTORY RECEPTOR CELLS** The *olfactory receptor cells* each have about a half dozen to a dozen little "hairs," called *cilia*, that project into the cavity. Like taste buds, there are receptor sites on these hair cells that send signals to the brain when stimulated by the molecules of substances that are in the air moving past them. Wait a minute---you mean that when I can smell something like a skunk, there are little particles of skunk odor IN my nose? Yes. When a person is sniffing something, the sniffing serves to move molecules of whatever the person is trying to smell into the nose and into the nasal cavities. That's okay when it's the smell of baking bread, apple pie, flowers, and the like, but when it's skunk, rotten eggs, dead animals---well, try not to think about it too much. Olfactory receptors are like taste buds in another way, too. Olfactory receptors also have to be replaced as they naturally die off, about every 5 to 8 weeks. Unlike the taste buds, there are way more than five types of olfactory receptors---in fact, there are at least 1,000 of them. You might remember from Chapter Two that signals from the olfactory receptors in the nasal cavity do not follow the same path as the signals from all the other senses. Vision, hearing, taste, and touch all pass through the thalamus and then on to the area of the cortex that processes that particular sensory information. But the sense of smell has its own special place in the brain---the olfactory bulbs, which are actually part of the brain. **THE OLFACTORY BULBS** The **olfactory bulbs** are located right on top of the sinus cavity on each side of the brain directly beneath the frontal lobes. (Refer back to Figure 3.11.) The olfactory receptors send their neural signals directly up to these bulbs, bypassing the thalamus, the relay center for all other sensory information. The olfactory information is then sent from the olfactory bulbs to higher cortical areas, including the primary olfactory cortex (the *piriform cortex*), the orbitofrontal cortex, and the amygdala (remember from Chapter Two that the orbitofrontal cortex and amygdala play important roles in emotion). to Chapter Two: The Biological Perspective, pp. 72 and 76. **Somesthetic Senses: What the Body Knows** So far, this chapter has covered vision, hearing, taste, and smell. That leaves touch. What is thought of as the sense of touch is really several sensations, originating in several different places in---and on---the body. It's really more accurate to refer to these as the body senses, or **somesthetic senses**. The first part of that word, *soma*, means "body," as mentioned in Chapter Two. The second part, *esthetic*, means "feeling," hence, the name. There are three somesthetic sense systems, the **skin senses** (having to do with touch, pressure, temperature, and pain), the **kinesthetic sense** (having to do with the location of body parts in relation to each other), and the **vestibular senses** (having to do with movement and body position). **PERCEPTION OF TOUCH, PRESSURE, AND TEMPERATURE** **3.7** What allows people to experience the sense of touch, pain, motion, and balance? Here's a good trivia question: What organ of the body is about 20 square feet in size? The answer is the skin. Skin is an organ. Its purposes include more than simply keeping bodily fluids in and germs out; skin also receives and transmits information from the outside world to the central nervous system (specifically, to the somatosensory cortex). to Chapter Two: The Biological Perspective, p. 75. Information about light touch, deeper pressure, hot, cold, and even pain is collected by special receptors in the skin's layers. **TYPES OF SENSORY RECEPTORS IN THE SKIN** There are about half a dozen different receptors in the layers of the skin. (See Figure 3.12.) Some of them will respond to only one kind of sensation. For example, the *Pacinian corpuscles* are just beneath the skin and respond to changes in pressure. There are nerve endings that wrap around the ends of the hair follicles, a fact people may be well aware of when they tweeze their eyebrows, or when someone pulls their hair. These nerve endings are sensitive to both pain and touch. There are *free nerve endings* just beneath the uppermost layer of the skin that respond to changes in temperature and to pressure---and to pain. Yes, there are pain nerve fibers in the internal organs as well as receptors for pressure. How else would people have a stomachache or intestinal\* pain---or get that full feeling of pressure when they've eaten too much or their bladder is full? There are actually different types of pain. There are receptors that detect pain (and pressure) in the organs, a type of pain called *visceral pain*. Pain sensations in the skin, muscles, tendons, and joints are carried on large nerve fibers and are called *somatic pain*. Somatic pain is the body's warning system that something is being, or is about to be, damaged and tends to be sharp and fast. Another type of somatic pain is carried on small nerve fibers and is slower and more of a general ache. This somatic pain acts as a kind of reminder system, keeping people from further injury by reminding them that the body has already been damaged. For example, if you hit your thumb with a hammer, the immediate pain sensation is of the first kind---sharp, fast, and bright. But later the bruised tissue simply aches, letting you know to take it easy on that thumb. People may not like pain, but its function as a warning system is vitally important. There are people who are born without the ability to feel pain, rare conditions called *congenital analgesia* and *congenital insensitivity to pain with anhidrosis (CIPA).* Children with these disorders cannot feel pain when they cut or scrape themselves, leading to an increased risk of infection when the cut goes untreated (Mogil, 1999). They fear nothing---which can be a horrifying trial for the parents and teachers of such a child. These disorders affect the neural pathways that carry pain, heat, and cold sensations. (Those with CIPA have an additional disruption in the body's heat--cold sensing perspiration system \[anhidrosis\], so that the person is unable to cool off the body by sweating.) A condition called *phantom limb pain* occurs when a person who has had an arm or leg removed sometimes "feels" pain in the missing limb (Nikolajsen & Jensen, 2001; Woodhouse, 2005). As many as 50 to 80 percent of people who have had amputations experience various sensations: burning, shooting pains, or pins-and-needles sensations where the amputated limb used to be. Once believed to be a psychological problem, some now believe that it is caused by the traumatic injury to the nerves during amputation (Ephraim et al., 2005). **PAIN: GATE-CONTROL THEORY** The best current explanation for how the sensation of pain works is called *gate-control* *theory*, first proposed by Melzack and Wall (1965) and later refined and expanded (Melzack & Wall, 1996). In this theory, the pain signals must pass through a "gate" located in the spinal cord. The activity of the gate can be closed by nonpain signals coming into the spinal cord from the body and by signals coming from the brain. The gate is not a physical structure but instead represents the relative balance in neural activity of cells in the spinal cord that receive information from the body and then send information to the brain. Stimulation of the pain receptor cells releases a chemical called *substance P* (for "pain," naturally). Substance P released into the spinal cord activates other neurons that send their messages through spinal gates (opened by the pain signal). From the \*intestinal: having to do with the tubes in the body that digest food and process waste material spinal cord, the message goes to the brain, activating cells in the thalamus, somatosensory cortex, areas of the frontal lobes, and the limbic system. The brain then interprets the pain information and sends signals that either open the spinal gates farther, causing a greater experience of pain, or close them, dampening the pain. Of course, this decision by the brain is influenced by the psychological aspects of the pain-causing stimulus. Anxiety, fear, and helplessness intensify pain, whereas laughter, distraction, and a sense of control can diminish it. (This is why people might bruise themselves and not know it if they were concentrating on something else.) Pain can also be affected by competing signals from other skin senses, which is why rubbing a sore spot can reduce the feeling of pain. Those same psychological aspects can also influence the release of the *endorphins*, the body's natural version of morphine. to Chapter Two: The Biological Perspective, p. 54*.* Endorphins can inhibit the transmission of pain signals in the brain, and in the spinal cord they can inhibit the release of substance. I've always heard that women are able to stand more pain than men. Is that true? On the contrary, research has shown that women apparently feel pain more intensely than do men, and they also report pain more often than men do (Chesterton et al., 2003; Faucett et al., 1994; Norrbrink et al., 2003). Men have been shown to cope better with many kinds of pain, possibly because men are often found to have a stronger belief than women that they can (or should) control their pain by their own efforts ( Jackson et al., 2002). **THE KINESTHETIC SENSE** Special receptors located in the muscles, tendons, and joints are part of the body's sense of movement and position in space---the movement and location of the arms, legs, and so forth in relation to one another. This sense is called *kinesthesia*, from the Greek words *kinein* ("to move") and *aesthesis* ("sensation"). When you close your eyes and raise your hand above your head, you know where your hand is because these special receptors, called proprioceptors, tell you about joint movement or the muscles stretching or contracting. If you have ever gotten sick from traveling in a moving vehicle, you might be tempted to blame these proprioceptors. Actually, it's not the proprioceptors in the body that make people get sick. The culprits are special structures in the ear that tell us about the position of the body in relation to the ground and movement of the head that make up the *vestibular sense*---the sense of balance. **THE VESTIBULAR SENSE** The name of this particular sense comes from a Latin word that means "entrance" or "chamber."The structures for this sense are located in the innermost chamber of the ear. There are two kinds of vestibular organs, the otolith organs and the semicircular canals. The *otolith organs* are tiny sacs found just above the cochlea. These sacs contain a gelatin-like fluid within which tiny crystals are suspended (much like pieces of fruit in a bowl of Jello®). The head moves and the crystals cause the fluid to vibrate, setting off some tiny hairlike receptors on the inner surface of the sac, telling the person that he or she is moving forward, backward, sideways, or up and down. (It's pretty much the way the cochlea works but with movement being the stimulus instead of sound vibrations.) The *semicircular canals* are three somewhat circular tubes that are also filled with fluid that will stimulate hairlike receptors when rotated. Having three tubes allows one to be located in each of the three planes of motion. Remember learning in geometry class about the *x*-, *y*-, and *z*-axes? Those are the three planes through which the body can rotate, and when it does, it sets off the receptors in these canals. When you spin around and then stop, the fluid in the horizontal canal is still rotating and will make you feel dizzy because your body is telling you that you are still moving, but your eyes are telling you that you have stopped. **MOTION SICKNESS** This disagreement between what the eyes say and what the body says is pretty much what causes *motion sickness*, the tendency to get nauseated when in a moving vehicle, especially one with an irregular movement. Normally, the vestibular sense coordinates with the other senses. But for some people, the information from the eyes may conflict a little too much with the vestibular organs, and dizziness, nausea, and disorientation are the result. This explanation of motion sickness is known as **sensory conflict theory** (Oman, 1990; Reason & Brand, 1975). The dizziness is the most likely cause of the nausea. Many poisons make a person dizzy, and the most evolutionarily adaptive thing to do is to expel the poison. Even without any poison in a case of motion sickness, the nausea occurs anyway (Treisman, 1977). One way some people overcome motion sickness is to focus on a distant point or object. This provides visual information to the person about how he or she is moving, bringing the sensory input into agreement with the visual input. This is also how ballerinas and ice skaters manage not to get sick when turning rapidly and repeatedly--- they focus their eyes at least once on some fixed object every so many turns. Astronauts, who travel in low gravity conditions, can get a related condition called space motion sickness (SMS). This affects about 60 percent of those who travel in space, typically for about the first week of space travel. After that time of adjustment, the astronauts are able to adapt and the symptoms diminish. Repeated exposure to some environment that causes motion sickness---whether it is space, a car, a train, or some other vehicle---is actually one of the best ways to overcome the symptoms (Hu & Stern, 1999). What are perception and perceptual constancies? **Perception** is the method by which the brain takes all the sensations people experience at any given moment and allows them to be interpreted in some meaningful fashion. Perception has some individuality to it. For example two people might be looking at a cloud and while one thinks it's shaped like a horse, the other thinks it's more like a cow. They both *see* the same cloud, but they *perceive* that cloud differently. As individual as perception might be, some similarities exist in how people perceive the world around them, as the following section will discuss. **THE CONSTANCIES: SIZE, SHAPE, AND BRIGHTNESS** One form of perceptual constancy\* is **size constancy** the tendency to interpret an object as always being the same size, regardless of its distance from the viewer (or the size of the image it casts on the retina). So if an object that is normally perceived to be about 6 feet tall appears very small on the retina, it will be interpreted as being very far away. Another perceptual constancy is the tendency to interpret the shape of an object as constant, even when it changes on the retina. This **shape constancy** is why a person still perceives a coin as a circle even if it is held at an angle that makes it appear to be an oval on the retina. Dinner plates on a table are also seen as round, even though from the angle of viewing they are oval. (See Figure 3.13.) A third form of perceptual constancy is **brightness constancy**, the tendency to perceive the apparent brightness of an object as the same even when the light conditions change. If a person is wearing black pants and a white shirt, for example, in broad daylight the shirt will appear to be much brighter than the pants. But if the sun is covered by thick clouds, even though the pants and shirt have less light to reflect than previously, the shirt will still appear to be just as much brighter than the pants as before---because the different amount of light reflected from each piece of clothing is still the same difference as before (Zeki, 2001) **THE GESTALT PRINCIPLES** Remember the discussion of the Gestalt theorists in Chapter One? Their original focus on human perception can still be seen in certain basic principles today, including the basic principles of the Gestalt tendency to group objects and perceive whole shapes. **3.9** What are the Gestalt principles of perception? **FIGURE--GROUND RELATIONSHIPS** Take a look at the drawing of the cube in Figure 3.14. Which face of the cube is in the front? Look again---do the planes and corners of the cube seem to shift as you look at it? This is called the "Necker cube." It has been around officially since 1832, when a Swiss scientist who was studying the structure of crystals first drew it in his published papers. The problem with this cube is that there are conflicting sets of depth cues, so the viewer is never really sure which plane or edge is in the back and which is in the front---the visual presentation of the cube seems to keep reversing its planes and edges. A similar illusion can be seen in Figure 3.15. In this picture, the viewer can switch perception back and forth from two faces looking at each other to the outline of a goblet in the middle. Which is the figure in front and which is the background? **Figure--ground** relationships refer to the tendency to perceive objects or figures as existing on a background. People seem to have a preference for picking out figures from backgrounds even as early as birth. The illusions in Figures 3.14 and 3.15 are **reversible figures**, in which the figure and the ground seem to switch back and forth. **PROXIMITY** Another very simple rule of perception is the tendency to perceive objects that are close to one another as part of the same grouping, a principle called **proximity**, or "nearness." (See Figure 3.16.) **SIMILARITY Similarity** refers to the tendency to perceive things that look similar as being part of the same group. When members of a sports team wear uniforms that are all the same color, it allows people viewing the game to perceive them as one group even when they are scattered around the field or court. **CLOSURE Closure** is the tendency to complete figures that are incomplete. A talented artist can give the impression of an entire face with just a few cleverly placed strokes of the pen or brush---the viewers fill in the details. **CONTINUITY** The principle of **continuity** is easier to see than it is to explain in words. It refers to the tendency to perceive things as simply as possible with a continuous pattern rather than with a complex, broken-up pattern. Look at Figure 3.16 for an example of continuity. Isn't it much easier to see the figure on the left as two wavy lines crossing each other than as the little sections in the diagrams to the right? **CONTIGUITY Contiguity** isn't shown in Figure 3.16 because it involves not just nearness in space but nearness in time also. Basically, contiguity is the tendency to perceive two things that happen close together in time as being related. Usually the first occurring event is seen as causing the second event. Ventriloquists\* make vocalizations without appearing to move their own mouths but move their dummy's mouth instead. The tendency to believe that the dummy is doing the talking is due largely to contiguity There is one other principle of perceptual grouping that was not one of the original principles. It was added to the list (and can be seen at the bottom of Figure 3.16) by Stephen Palmer (Palmer, 1992). In *common region*, the tendency is to perceive objects that are in a common area or region as being in a group. In Figure 3.16, people could perceive the stars as one group and the circles as another on the basis of similarity. But the colored backgrounds so visibly define common regions that people instead perceive three groups---one of which has both stars and circles in it. **DEPTH PERCEPTION** **3.10** What is depth perception and what kind of cues are important for it to occur? The capability to see the world in three dimensions is called **depth perception**. It's a handy ability because without it you would have a hard time judging how far away objects are. How early in life do humans develop depth perception? It seems to develop very early in infancy, if it is not actually present at birth. People who have had sight restored have almost no ability to perceive depth if they were blind from birth. Depth perception, like the constancies, seems to be present in infants at a very young age. to Chapter Eight: Development Across the Life Span, pp. 310-311*.* Various cues exist for perceiving depth in the world. Some require the use of only one eye (**monocular cues**) and some are a result of the slightly different visual patterns that exist when the visual fields\* of both eyes are used (**binocular cues**). **MONOCULAR CUES** Monocular cues are often referred to as **pictorial depth cues** because artists can use these cues to give the illusion of depth to paintings and drawings. Examples of these cues are discussed next and can be seen in Figure 3.17. 1\. **Linear perspective:** When looking down a long interstate highway, the two sides of the highway appear to merge together in the distance. This tendency for lines that are actually parallel to *seem* to converge\*\* on each other is called **linear perspective**. It works in pictures because people assume that in the picture, as in real life, the converging lines indicate that the "ends" of the lines are a great distance away from where the people are as they view them. 2\. **Relative size:** The principle of size constancy is at work in **relative size**, when objects that people expect to be of a certain size appear to be small and are, therefore, assumed to be much farther away. Movie makers use this principle to make their small models seem gigantic but off in the distance. **Overlap:** If one object seems to be blocking another object, people assume th the blocked object is behind the first one and, therefore, farther away. This cue is also known as **interposition**. **Aerial (atmospheric) perspective:** The farther away an object is, the hazier th object will appear to be due to tiny particles of dust, dirt, and other pollutants in the air, a perceptual cue called **aerial (atmospheric) perspective**. This is why dis tant mountains often look fuzzy, and buildings far in the distance are blurrier than those that are close. **Texture gradient:** If there are any large expanses of pebbles, rocks, or patterned roads (such as a cobblestone street) nearby, go take a look at them one day. The pebbles or bricks that are close to you are very distinctly textured, but as you look farther off into the distance, their texture becomes smaller and finer. **Texture gra** **dient** is another trick used by artists to give the illusion of depth in a painting. **Motion parallax:** The next time you're in a car, notice how the objects outside the car window seem to zip by very fast when they are close to the car, and objects in the distance, such as mountains, seem to move more slowly. This dis crepancy in motion of near and far objects is called **motion parallax**.. **Accommodation:** A monocular cue that is not one of the pictorial cues, **accommodation** makes use of something that happens inside the eye. The len of the human eye is flexible and held in place by a series of muscles. The discu sion of the eye earlier in this chapter mentioned the process of visual accommo dation as the tendency of the lens to change its shape, or thickness, in response to objects near or far away. The brain can use this information about accommo dation as a cue for distance. Accommodation is also called a "muscular cue." **OCULAR CUES** As the name suggests, these cues require the use of two eyes. **Convergence:** Another muscular cue, **convergence**, refers to the rotation of the two eyes in their sockets to focus on a single object. If the object is close, the convergence is pretty great (almost as great as crossing the eyes). If the object is far, the convergence is much less. Hold your finger up in front of your nose, and then move it away and back again. That feeling you get in the mus cles of your eyes is convergence. (See Figure 3.18, left.) **Binocular disparity: Binocular disparity** is a scientific way of saying that because the eyes are a few inches apart, they don't see exactly the same image. The brain interprets the images on the retina to determine distance from the eyes. If the two images are very different, the object must be pretty close. If they are almost identical, the object is far enough away to make the retinal disparity very small. You can demonstrate this cue for yourself by holding an object in front of your nose. Close one eye, note where the object is, and then open that eye and close the other. There should be quite a difference in views. But if you do the same thing with an object that is across the room, the image doesn't seem to "jump" or move nearly as much, if at all. (See Figure 3.18, right.) In spite of all the cues for perception that exist, even the most sophisticated perceiver can still fail to perceive the world as it actually is, as the next section demonstrates. **PERCEPTUAL ILLUSIONS** You've mentioned the word illusion several times. Exactly what are illusions, and why is it so easy to be fooled by them? An *illusion* is a perception that does not correspond to reality: People *think* they see something when the reality is quite different. Another way of thinking of illusions is as visual stimuli that "fool" the eye. (Illusions are not hallucinations: an illusion is a distorted perception of something that is really there, but a hallucination originates in the brain, not in reality.) **3.11** What are visual illusions and how can they and other factors influence and alter perception? Research involving illusions can be very useful for both psychologists and neuroscientists. These studies often provide valuable information about how the sensory receptors and sense organs work and how humans interpret sensory input. Sometimes illusions are based on early sensory processes, subsequent processing, or higher level assumptions made by the brain's visual system (Eagleman, 2001; Macknik et al., 2008). We've already discussed one visual illusion, color afterimages, which are due to opponent-processes in the retina or lateral geniculate nucleus (LGN) of the thalamus after light information has been detected by the rods and cones. Another postdetection, but still rather early, process has been offered for yet another illusion. **THE HERMANN GRID** Look at the matrix of squares in Figure 3.19. Notice anything interesting as you look at different parts of the figure, particularly at the intersections of the white lines? You probably see gray blobs or diamonds that fade away or disappear completely when you try to look directly at them.This is the Hermann grid. One explanation for this illusion is attributed to the responses of neurons in the primary visual cortex that respond best to bars of light of a specific orientation (Schiller & Carvey, 2005). Such neurons are called "simple cells" and were first discovered by David Hubel and Torsten Wiesel (Hubel & Wiesel, 1959). Hubel and Wiesel were later awarded the Nobel Prize for extensive work in the visual system. Other research into the Hermann grid illusion has documented that straight edges are necessary for this illusion to occur, as the illusion disappears when the edges of the grid lines are slightly curved, like a sine wave, and further suggests that the illusion may be due to a unique function of how our visual system processes information (Geier et al., 2008). **MÜLLER-LYER ILLUSION** One of the most famous visual illusions, the **Müller-Lyer** **illusion**, is shown in Figure 3.20. The distortion happens when the viewer tries to determine if the two lines are exactly the same length. They are identical, but one line looks longer than the other. (It's always the line with the angles on the end facing outward.) Why is this illusion so powerful? The explanation is that most people live in a world with lots of buildings. Buildings have corners. When a person is outside a building, the corner of the building is close to that person, while the walls seem to be moving away (like the line with the angles facing inward). When the person is inside a building, the corner of the room seems to move away from the viewer while the walls are coming closer (like the line with the angles facing outward). In their minds, people "pull" the inward-facing angles toward them like the outside corners of a building, and they make the outward-facing angles "stretch" away from them like the inside corners of the room (Enns & Coren, 1995; Gregory, 1990). Segall and colleagues (Segall et al., 1966) found that people in Western cultures, having carpentered buildings with lots of straight lines and corners (Segall and colleagues refer to this as a "carpentered world"), are far more susceptible to this illusion than people from non-Western cultures (having round huts with few corners---an "uncarpentered world"). Gregory (1990) found that Zulus, for example, rarely see this illusion. They live in round huts arranged in circles, use curved tools and toys, and experience few straight lines and corners in their world. **THE MOON ILLUSION** Another common illusion is the *moon illusion*, in which the moon on the horizon\* appears to be much larger than the moon in the sky (Plug & Ross, 1994). One explanation for this is that the moon high in the sky is all alone, with no cues for depth surrounding it. But on the horizon, the moon appears behind trees and houses, cues for depth that make the horizon seem very far away. The moon is seen as being behind these objects and, therefore, farther away from the viewer. Because people know that objects that are farther away from them yet still appear large are very large indeed, they "magnify" the moon in their minds---a misapplication of the principle of size constancy. This explanation of the moon illusion is called the *apparent distance hypothesis.* This explanation goes back to the second century A.D., first written about by the Greek-Egyptian astronomer Ptolemy and later further developed by an eleventh-century Arab astronomer, Al-Hazan (Ross & Ross, 1976). **ILLUSIONS OF MOTION** Sometimes people perceive an object as moving when it is actually still. One example of this takes place as part of a famous experiment in conformity called the *autokinetic effect*. In this effect, a small, stationary light in a darkened room will appear to move or drift because there are no surrounding cues to indicate that the light is *not* moving. Another is the *stroboscopic motion* seen in motion pictures, in which a rapid series of still pictures will seem to be in motion. Many a student has discovered that drawing little figures on the edges of a notebook and then flipping the pages quickly will also produce this same illusion of movement. Another movement illusion related to stroboscopic motion is the *phi phenomenon*, in which lights turned on in sequence appear to move. For example, if a light is turned on in a darkened room and then turned off, and then another light a short distance away is flashed on and off, it will appear to be one light moving across that distance. This principle is used to suggest motion in many theater marquee signs, flashing arrows indicating direction that have a series of lights going on and off in a sequence, and even in strings of decorative lighting, such as the "chasing" lights seen on houses at holiday times. What about seeing motion in static images? There are several examples, both classic and modern, of illusory movement or apparent motion being perceived in a static image. The debate about the causes for such illusions, whether they begin in the eyes or the brain, has been going on for at least 200 years (Troncoso et al., 2008). Look at Figure 3.21. What do you see? The "Rotating Snakes" illusion is one of many motion-illusion images designed by Dr. Akiyoshi Kitaoka. There have been a variety of explanations for this type of motion illusion, ranging from factors that depend on the image's luminance and/or the color arrangement, or possibly slight differences in the time it takes the brain to process this information. When fMRI and equipment used to track eye movements was used to investigate participants' perception of the illusion, researchers found that there was an increase in brain activity in a visual area sensitive to motion. However, this activity was greatest when accompanied by guided eye movements, suggesting eye movements play a significant role in the perception of the illusion (Kuriki et al., 2008). Eye movements have also been found to be a primary cause for the illusory motion seen in images based on a 1981 painting by Isia Levant, *The Enigma*. Look at the center of Figure 3.22, notice anything within the green rings? Many people will see the rings start to "sparkle" or the rings rotating. Why does this occur? By using special eye-tracking equipment that allowed them to record even the smallest of eye movement, researchers found that tiny eye movements called *microsaccades*, discussed earlier in the chapter, are directly linked to the perception of motion in *Enigma* and are at least one possible cause of the illusion (Troncoso et al., 2008). These two studies highlight some of the advances researchers have made in examining questions related to visual perception. For more information about the study of visual illusions as used in magic, and the study of such illusions from a neuroscientific perspective, see the Applying Psychology section at the end of the chapter. **OTHER FACTORS THAT INFLUENCE PERCEPTION** Human perception of the world is obviously influenced by things such as culture and misinterpretations of cues. Following are other factors that cause people to alter their perceptions. **PERCEPTUAL SETS AND EXPECTANCIES** People often misunderstand what is said to them because they were expecting to hear something else. People's tendency to perceive things a certain way because their previous experiences or expectations influence them is called **perceptual set** or **perceptual expectancy**. Although expectancies can be useful in interpreting certain stimuli, they can also lead people down the wrong path. For example, look at Figure 3.23. The drawing in the middle is a little hard to identify. People who look at these five drawings and start with the drawing on the far left (which is clearly a man's face) tend to see the middle drawing as a man's face. But people who begin looking from the far right (where the drawing is a kneeling woman with one arm over her chest and one touching her knee) see the middle picture as a woman. What you see depends on what you expect to see. The way in which people *interpret* what they perceive can also influence their perception. For example, people can try to understand what they perceive by using information they already have (as is the case of perceptual expectancy). But if there is no existing information that relates to the new information, they can look at each feature of what they perceive and try to put it all together into one whole. Anyone who has ever worked on a jigsaw puzzle knows that it's a lot easier to put it together if there is a picture of the finished puzzle to refer to as a guide. It also helps to have worked the puzzle before---people who have done that already know what it's going to look like when it's finished. In the field of perception, this is known as **topdown processing**---the use of preexisting knowledge to organize individual features into a unified whole. This is also a form of perceptual expectancy. If the puzzle is one the person has never worked before or if that person has lost the top of the box with the picture on it, he or she would have to start with a small section, put it together, and keep building up the sections until the recognizable picture appears. This analysis of smaller features and building up to a complete perception is called **bottom-up processing** (Cave & Kim, 1999). In this case, there is no expectancy to help organize the perception, making bottom-up processing more difficult in some respects. Fortunately, the two types of processing are often used together in perceiving the surrounding world. Would people of different cultures perceive objects differently because of different expectancies? Some research suggests that this is true. For example, take a look at Figure 3.24. This figure is often called the "devil's trident." Europeans and North Americans insist on making this figure three dimensional, so they have trouble looking at it---the figure is impossible if it is perceived in three dimensions. But people in less technologically oriented cultures have little difficulty with seeing or even reproducing this figure, because they see it as a two-dimensional drawing, quite literally a collection of lines and circles rather than a solid object (Deregowski, 1969). By contrast, if you give Europeans and North Americans the task of reproducing a drawing of an upside-down face, their drawings tend to be more accurate because the upside-down face has become a "collection of lines and circles." That is, they draw what they actually see in terms of light and shadow rather than what they "think" is there three dimensionally. **Applying Psychology to Everyday Life:** **Beyond "Smoke and Mirrors"---The Psychological** **Science and Neuroscience of Magic** Many people enjoy watching magic acts in person or on television. Perhaps you have been amazed by a Mindfreak® performed by Criss Angel or the performance and edgy antics of Penn & Teller. If you are one of those people, you likely witnessed a performance that included many various illusions. And like many of us, you probably wondered at some point in the performance, "How did they do that?!" Did you think the tricks were due to some type of special device (such as a fake thumb tip for hiding a scarf ), or perhaps they were accomplished with "smoke and mirrors," or maybe the magician distracted the audience with one movement while actually doing something else to pull off the illusion? Magicians use many techniques to take advantage of, or manipulate, our actual level of awareness of what is happening right in front of us or perhaps to manipulate our attention. Though magic is not a new topic of interest in psychology, there has been renewed interest in recent years, especially in the neuroscientific study of magic. This view suggests that researchers can work alongside magicians so we may be able to gain a better understanding of various cognitive and perceptual processes by not only examining the sensory or physical mechanics behind magic tricks, or even the psychological explanations, but to look further by examining what is happening in the brain (Macknik & Martinez-Conde, 2009). Dr. Stephen L. Macknik and Dr. Susanna Martinez-Conde of the Barrow Neurological Institute are two neuroscientists who have teamed up with professional magicians to study their techniques and tricks in the effort to better understand the brain mechanisms underlying the illusions and how that information can be used by researchers in the laboratory. They have identified several types of illusions that can be used alone or in combination with others to serve as a basis for various magic tricks; two of these are visual illusions and cognitive illusions (Macknik et al., 2008). As discussed earlier in the chapter, visual illusions occur when our individual perception does not match a physical stimulus. These illusions are caused by organizational or processing biases in the brain. Furthermore, our brain activity from the perception does not directly match the brain activity associated with the physical stimulus (Macknik et al., 2008). One example Dr. Macknik and Dr. Martinez-Conde point out is similar to a trick you may have performed yourself in grade school. Did you ever take a pencil or pen, grasp it in the middle, and then shake or wiggle it up and down? If you did it correctly, the pen or pencil would appear to bend or be made of rubber. Magicians use this illusion when they "bend" solid objects, such as spoons. So what is the brain explanation? We have special neurons in the visual cortex that are sensitive to both motion and edges called *end-stopped neurons*. These neurons respond differently if an object is bouncing or moving up and down quickly, causing us to perceive a solid spoon or pencil as if it is bending. Another effect or trick that is based on the functioning of our visual system is when a magician makes an object disappear, such as a ball vanishing into the air or perhaps the outfit of an assistant changing suddenly. By showing the audience the target object, such as the ball or outfit, and then removing it very quickly from the visual field, the *persistence of vision* effect will make it appear that the object is still there. This is due to a response in vision neurons called the after-discharge, which will create an afterimage that lasts for up to 100 milliseconds after a stimulus is removed (Macknik et al., 2008). Again, you may have performed a similar trick if you have ever taken a lit sparkler or flashlight and twirled it around quickly to make a trail of light in the dark