Chapter 5: Genetics PDF
Document Details
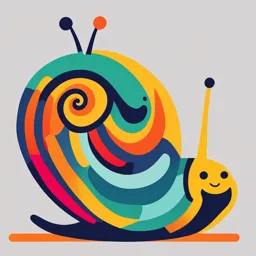
Uploaded by PromisedCouplet6066
Tags
Summary
This chapter introduces basic genetics for understanding modern medicine, such as antibiotic resistance and personalized therapies. It covers topics like genotype/phenotype relationships, how genes are organized in eukaryotic and prokaryotic cells, and the structure of DNA and RNA. The summary aims to introduce readers to foundational biological principles about cellular genetics.
Full Transcript
5 Genetics What Will We Explore? This chapter introduces you to basic genetics topics that are essential for understanding a number of concepts in modern medicine, including antibiotic resistance, bacterial growth, modes of drug action, personalized medicine, gene therapy, and the development of the...
5 Genetics What Will We Explore? This chapter introduces you to basic genetics topics that are essential for understanding a number of concepts in modern medicine, including antibiotic resistance, bacterial growth, modes of drug action, personalized medicine, gene therapy, and the development of therapies to treat viral epidemics, from HIV to Ebola to Zika. Why Is It Important? Genes impact our risk for diseases and how we respond to drugs. Even our likelihood for infection is dictated by our genetics. For example, an extremely rare genetic alteration protects a very small segment of the population against HIV infection. Of course, not all genetic changes confer an advantage; at least 6,000 medical disorders, CLINICAL CASE including cystic fibrosis and sickle cell anemia, result from genetic changes. Genetics is also propelling us toward an age of personalized medicine that promises customized preventive therapies, earlier diagnosis, and tapered treatments that cure us based on our individual genetic makeup. Hundreds of genes are already associated with specific diseases, and the effectiveness of at least a dozen drugs has been studied in the context of patient genetic profiles. In recognition of the increasing role of genetics in medical therapies, the FDA has outlined the standards for using genetic information for personalized medicine and a number of FDA-approved genetic-based theraThe Case of the NCLEX Spreading Superbug pies are in use. In the future, healthHESI care providers will increasingly rely How can we track and TEAS prevent the spread of on genetic profiles to diagnose and a bacterium from an treat patients. Thus it is essential isolation ward? that the healthcare team of tomorrow develops a working knowledge Scan this code or visit the Mastering Microbiology Study of basic principles in genetics and Area to watch the case and find links these principles to patient out how genetics can explain this medical mystery. care. 121 M05_NORM8290_01_SE_C05.indd 121 24/08/17 10:15 AM HEREDITY BASICS After reading this section, you should be able to: 1 Define genotype and phenotype and discuss their relationship. 2 Compare and contrast how genetic material is organized in eukaryotic and prokaryotic cells. 3 Describe the structural features of DNA and RNA nucleotides. 4 Relate the directionality of nucleotides to the formation of phosphodiester bonds. 5 Describe the structural features of RNA and state how RNA differs from DNA. 6 Explain the flow of genetic material as it is presented by the “central dogma.” Genotype determines phenotype. The modern field of genetics studies genes, their function, and how variations arise in genomes—the entire collection of genetic material in a cell or virus. Gregor Mendel, an Austrian monk who lived in the mid-1800s, is often called the father of modern genetics. His pioneering work with pea plants paved the way for us to understand that traits are heritable, or passed from one generation to the next. By the early 1900s the term gene was used to describe heritable units of genetic material that determine a particular trait. The theory that the genetic makeup, or genotype, of an organism influences its physiological and physical traits (phenotype) then became widely accepted. You can think of genomes as instruction manuals that determine all the possible features of a cell or virus. Cells have deoxyribonucleic acid (DNA) genomes, while viruses have either DNA or ribonucleic acid (RNA) genomes (FIG. 5.1). This chapter focuses on cellular genetics. (See Chapter 6 for viruses.) DNA and RNA were first introduced in Chapter 2, but here you will learn more about the structure and function of these molecules that are the language of life. Let’s begin by reviewing DNA as the substance of cellular genomes and then we’ll explore how DNA is built and read. Prokaryotic and eukaryotic genomes differ in size and organization. CHEM NOTE DNA and RNA are nucleic acids made of repeating subunits called nucleotides. Nucleotides are made up of a phosphate, a sugar (deoxyribose in DNA/ribose in RNA), and one of five possible nitrogen bases (A, T, G, C, or U). In general, the more complex an organism, the more genes it has. Prokaryotic cells tend to have smaller genomes compared with eukaryotic cells, although there is amazing variety in the sizes of genomes among organisms. For example, the nonpathogenic bacterium E. coli strain K12 has around 4,400 genes, while a pathogenic strain of E. coli called O157:H7 has about 5,500 genes. For a eukaryotic comparison, consider that a yeast cell has about 6,000 genes, while a human cell has about 24,000 genes. FIGURE 5.1 Genomes The genome is all the genetic material in a cell or virus. Illustrations are not to scale. DNA or RNA Chromosomal DNA Mitochondrial DNA Plasmid DNA Chloroplastic DNA (only in photosynthetic cells) Viral genome DNA or RNA 122 Prokaryotic genome Eukaryotic genome DNA ONLY CHAPTER 5 Genetics M05_NORM8290_01_SE_C05.indd 122 24/08/17 10:15 AM All cells organize their genomes into chromosomes, which are carefully packaged strands of DNA associated with organizational proteins. The number of chromosomes does not influence organism sophistication, or even its total number of genes. For example, human cells have 46 chromosomes; chimpanzee cells have 48; and single-celled amoebas have hundreds of chromosomes. Most prokaryotic cells organize their genome in one to three chromosomes located in the nucleoid region of the cell (recall that prokaryotic cells lack a nucleus). Most bacteria have a single circular chromosome, but Streptomyces species, many of which make useful antibiotics, and the bacterium that causes Lyme disease, Borrelia burgdorferi, are examples of prokaryotes with linear chromosomes. In contrast, eukaryotic cells tend to have numerous linear chromosomes housed in the cell’s nucleus. Eukaryotic chromosomes also have organizational proteins called histones to help keep their DNA from getting tangled. Just as thread is wound around a spool to keep it organized, DNA wraps around these histones. Prokaryotes also make use of several histone-like proteins to organize their DNA into chromosomes. While the bulk of a cell’s genome is found in chromosomes, prokaryotic and eukaryotic genomes also may include plasmids, which are pieces of DNA that tend to be circular and exist outside of the chromosomal DNA. Plasmids usually confer a survival advantage to a cell, including genes related to antibiotic resistance, among other things. Mitochondria and chloroplasts also contribute to eukaryotic genomes since these organelles have their own DNA. The prokaryote-like features of mitochondrial and chloroplastic genomes suggest that these organelles evolved from a bacterial ancestor. (See Chapter 4 for more on endosymbiotic theory). TABLE 5.1 summarizes key differences between prokaryotic and eukaryotic genomes. The sequencing of the human genome was completed in 2003 and took 13 years and about a billion dollars to complete. Now a person can have his or her entire genome sequenced in a few days for about $1,000. Likewise, researchers have decoded the genomes of thousands of other organisms and viruses so far. Our progress in genomics, a specialized area in genetics that focuses on characterizing entire genomes, occurred rapidly, considering that the structure of DNA was not confirmed until the mid-1950s. TABLE 5.1 Genome Characteristics Factor Prokaryotic Genomes Eukaryotic Genomes Complexity: Simple More complex Genome can include: Chromosomal DNA and plasmids Chromosomal DNA, DNA in mitochondria/ chloroplasts, and plasmids Chromosomes: Few (generally only one); usually circular Many; nuclear chromosomes are linear Location of chromosome(s): Nucleoid region Nucleus DNA organized by: Histone-like proteins Histones CHEM NOTE Numbering carbons in DNA and RNA All nucleotides have a five-carbon sugar. The first carbon in the sugar is called the 1’ (“one prime”) carbon; the second is the 2’ carbon, and so on. The 1’ carbon links to a nitrogen base, the 3’ carbon always bonds to a hydroxyl (OH) group, and the 5’ carbon links to phosphate. Phosphate Nitrogen base Sugar Deoxyribose in DNA Ribose in RNA 5´ The nucleic acids DNA and RNA govern cell life. The nucleic acids DNA and RNA collaborate to govern all aspects of cell life, from structure to function. O 1´ 4´ 3´ DNA’s Structure and Function 2´ OH Nucleic acids are built from nucleotides, which have three basic parts: a phosphate, a sugar (deoxyribose in DNA or ribose in RNA), and one nitrogen base (adenine (A), guanine (G), cytosine (C), thymine (T), or uracil (U)) (FIG. 5.2). The five nitrogen bases are classified as either purines or pyrimidines, depending on their chemical structure (see TABLE 5.2). The nitrogen bases in nucleic acids pair up according to specific rules, where A bonds with T or U (AT pairs in DNA; H (OH in ribose) Nitrogen bases can be N N N TABLE 5.2 DNA and RNA Nitrogen Bases Nitrogen Base Family Pairs with Found in Adenine (A) Purine Thymine ( T ) DNA and RNA Guanine (G) Purine Cytosine (C) DNA and RNA Cytosine (C) Pyrimidine Guanine (G) DNA and RNA Thymine (T) Pyrimidine Adenine (A) Only DNA Uracil (U) Pyrimidine Adenine (A) Only RNA N OR N Pyrimidines Thymine (T) DNA only Uracil (U) RNA only Cytosine (C) H N N Purines Adenine (A) Guanine (G) FIGURE 5.2 Nucleotides Nucleotides make up DNA and RNA. Heredity Basics M05_NORM8290_01_SE_C05.indd 123 123 24/08/17 10:15 AM Sugar– phosphate backbone “rails” Nitrogen base pair “rungs” 5ʹ Sugar T A G C C Phosphate T Nucleotide G A 3ʹ 3ʹ Hydrogen bond AU pairs in RNA) and G bonds with C (GC pairs in DNA and RNA). The bases can occur in any sequence, meaning there is a limitless “code” to store information. The nucleotide sequence of DNA encodes the information that cells need to survive. DNA is a long, double-stranded molecule that curves into a helix; in many ways it resembles a twisted ladder (FIG. 5.3). DNA’s “rungs” consist of the nitrogen bases (A, T, G, and C). The set pairings of the bases ensure that there is always a purine across from a pyrimidine, so that DNA has a consistent and predictable dimensionality, even though it has an unpredictable sequence of bases. Base-pairing rules also establish that one strand of DNA directs what the partner strand looks like; therefore, the strands are said to be complementary of each other, or balanced. The “side rails” of the DNA molecule “ladder” are an alternating pattern of sugar and phosphate molecules held together by phosphodiester bonds (FIG. 5.4). These covalent bonds give DNA strands directionality—just as we read from left to right, DNA is built and read with a specific orientation. DNA has 5’ to 3’ directionality (pronounced “five-prime to three-prime”) because it is built by linking a 5’ phosphate to a 3’ hydroxyl group. FIG. 5.5 presents an analogy to help you understand DNA’s chemical directionality. Much like two lanes of traffic headed in the opposite direction, doublestranded DNA has an antiparallel arrangement. In DNA one strand runs 5’ to 5ʹ In nucleotides, a base (A, T, G, or C) is attached to the 1´, or first carbon of a sugar. A hydroxyl group (OH) is attached to the 3´ carbon and a phosphate is attached to the 5´ carbon. Phosphate O 4´ C H FIGURE 5.3 DNA’s structure DNA has an antiparallel arrangement. The sugar–phosphate backbone makes up the “rails” of the DNA ladder while the nitrogen bases form the “rungs” of the ladder. Note that T pairs with A and G pairs with C. 5´ CH2 5´ CHEM NOTE 5´ 5´ 3´ 3´ OH Phosphodiester bonds are covalent bonds that link nucleotides together in DNA and RNA. H Phosphodiester bond 3´ Base H 2´ C 3´C OH Critical Thinking What would the 5’–3’ sugar–phosphate backbone look like if DNA had a parallel arrangement instead of antiparallel? 1´ C Sugar 5´ 5´ 5´ 3´ 3´ 3´ OH 1 The structure of DNA resembles a twisted ladder. DNA is built from 5´ to 3´ because the 5´ phosphate of one nucleotide bonds to the 3´ hydroxyl (OH) group of an adjacent nucleotide. 2 5´ Phosphodiester bonds link nucleotides together to form the sugar–phosphate “backbone” of DNA. 3´ OH 3 Because DNA is built by 5´ to 3´ phosphodiester bonds, no matter how long the molecule is, one end is always a 5´ phosphate and the opposite end is always a 3´ hydroxyl group. FIGURE 5.4 Phosphodiester bonds 124 CHAPTER 5 Genetics M05_NORM8290_01_SE_C05.indd 124 24/08/17 10:15 AM 3’ and the partner strand runs 3’ to 5’. Antiparallel directionality allows the complementary base pairs of DNA to properly associate, much like the cogs of a gear have to be properly positioned to interlock. RNA’s Structure and Function The nucleotides in RNA are called ribonucleotides, which are built using the sugar ribose, as opposed to the deoxyribose sugar found in DNA’s nucleotides. Some nitrogen bases found in RNA are also a little different from those in DNA––in RNA, uracil (U) replaces thymine (T), leading to AU base pairs in RNA instead of AT base pairs. Like DNA, RNA also has 5’ to 3’ directionality. Unlike DNA, which tends to exist primarily as a double-stranded helix, RNA is often single stranded. However, it does fold in on itself to form helical and loop structures. Types of RNA include messenger RNA, transfer RNA, and ribosomal RNA. All of them participate in making proteins. Later, in the protein synthesis section (see page 130), we will review their structures and functions. CHEM NOTE Phosphate groups are noted as PO43−. Hydroxyl groups are designated as R–OH, where R is often a carbon-based molecule. Hydroxyl groups are different from hydroxide ions (OH−). Genetic information typically flows from DNA, to RNA, to protein. The general flow of genetic information is usually from DNA to RNA to protein. That is, DNA directs production of RNA, and RNA then directs assembly of proteins, which are central to most cellular functions. This flow of information is sometimes called the central dogma of molecular biology.1 We now know that the flow of genetic information is not strictly from DNA to RNA and then to protein. As a result, the “central dogma” has been revised to take into account that 5ʹ Phosphate 5ʹ 5ʹ 3ʹ 3ʹ 5ʹ 3ʹ OH 5ʹ 5ʹ 3ʹ 3ʹ 3ʹ 1 Imagine a paper clip where half the clip is yellow and half the clip is purple. The yellow half represents the 5ʹ phosphate and the purple half represents the 3ʹ hydroxyl group. 2 Imagine the purple end of one paper clip can only link to the yellow end of another paper clip. 3 No matter how long the chain, one end will be yellow and the other will be purple. 4 If you made two separate paper clip chains you could lay them antiparallel to each other. 3ʹ OH 5 5ʹ Phosphate DNA works like these paper clip chains. Nucleotides are added in a 5 ʹ to 3ʹ manner so that no matter how long the DNA strand is, one end will be a 5ʹ phosphate and the other end will be a 3ʹ hydroxyl group. FIGURE 5.5 Chemical directionality of DNA 1 The term “dogma” means an unquestionably true principle—something that really has no place in science, which embraces questioning to advance understanding. Scientist Francis Crick reportedly coined the phrase “central dogma” without truly understanding the definition of the word—he just thought it was catchy. The phrase persists in scientific literature and as such, is included here for historical perspective. Heredity Basics M05_NORM8290_01_SE_C05.indd 125 125 24/08/17 10:15 AM RNA can be used as a template to make DNA and that the flow of genetic information is not as unidirectional as was previously envisioned (FIG. 5.6). We will discuss this modified flow of genetic information further when we cover transcription (see page 130). Transcription Translation Reverse transcription DNA 3D model of DNA RNA Protein FIGURE 5.6 Flow of genetic information The primary flow of genetic information in cells is from DNA to RNA to protein; this is referred to as the “central dogma” of molecular biology. Critical Thinking RNA could be considered a more versatile nucleic acid than DNA. What information in this figure supports that idea? TRAINING TOMORROW’S HEALTH TEAM Purines and Gout In humans, uric acid is the product of metabolizing dietary purines. When uric acid accumulates in the blood (hyperuricemia) it may cause gout, a painful form of arthritis in which uric acid crystallizes and settles in joints—especially joints of the extremities—causing inflammation and pain. The small joint at the base of the big toe is the most commonly affected, followed by knees and ankles. Gout tends to be more common in men than in women, in part because men have higher levels of uric acid in their blood than women. There is also a genetic component to gout. People with gout should follow a diet that is low in meat, poultry, shellfish, and fish—–all of which have high purine content. However, dairy products are a great way for gout patients to meet their protein needs while minimizing dietary purines. In addition to diet management to reduce hyperuricemia, medications like allopurinol (Aloprim, Zyloprim, Lopurin, etc.) or febuxostat (Uloric) are often prescribed. Q UE STIO N 5. 1 Why do you think meat contains higher levels of purines than dairy products? 126 CHAPTER 5 Genetics M05_NORM8290_01_SE_C05.indd 126 24/08/17 10:15 AM BUILD YOUR FOUNDATION 1. What is the primary difference between genotype and phenotype? 2. List the various sources of genomic material in eukaryotes and prokaryotes. 3. Compare and contrast how DNA is packaged in prokaryotic and eukaryotic cells. 4. Describe the building blocks of nucleic acids and describe how they differ between DNA and RNA. 5. Explain how DNA’s chemical 5´ to 3´ directionality is established. 6. What are the structural and functional characteristics of DNA and RNA and how do they compare to each other? 7. What does the “central dogma” state? QUICK QUIZ Build your foundation by answering the Quick Quiz: scan this code or visit the Mastering Microbiology Study Area to quiz yourself. DNA REPLICATION DNA replication allows cells to copy their DNA. DNA replication is the process by which a cell copies its genome before it divides. In general, DNA replication involves unwinding the original DNA, copying it, and then rewinding the parent DNA and the newly made DNA. To make a new DNA strand, a typical bacterial cell like E. coli has to copy about four million nucleotides, while a human cell has to copy about three billion nucleotides. To give you an idea of the enormity of these numbers, if our genome was written out and you read one nucleotide per second, it would take you just over 101 years to read the entire sequence out loud without stopping.2 Fortunately, DNA replication is incredibly fast and accurate; relatively few mutations (errors) are made. For example, a bacterium like E. coli can copy about 500 nucleotides per second and makes an error only about once every 10–100 billion base pairs.3 This high accuracy rate is due to complementary base-pairing rules, as well as proofreading mechanisms of certain DNA replication enzymes. While there are definite differences in how prokaryotic and eukaryotic cells replicate DNA, they also have a lot in common regarding the process. The next section focuses on how the bacterium E. coli copies its DNA. Enzymes are proteins that facilitate chemical reactions in a cell. There are a number of enzymes associated with DNA replication (TABLE 5.3). In E. coli, at least five different DNA polymerases have been characterized, although only two of the five are key participants in DNA replication; the rest tend to have a role in fixing damaged DNA. (For more details on enzymes, see Chapter 8.) After reading this section, you should be able to: 7 Discuss the role of DNA replication and factors that contribute to its accuracy. 8 Name the enzymes required for DNA replication and discuss their specific functions. 9 Discuss how DNA replication begins. 10 Describe how the directionality of DNA impacts replication. 11 Compare and contrast DNA replication on the leading and lagging strands. 12 Highlight differences between how prokaryotic and eukaryotic cells conduct DNA replication. Starting DNA Replication Just as you have to open a book before photocopying it, the DNA helix must be opened before duplication occurs. In prokaryotes, DNA replication starts when a collection of factors called the primosome is recruited to a specific point in the prokaryotic chromosome called the origin of replication. The primosome includes helicase to unwind the DNA and primase to lay down RNA primers to jumpstart replication. 2 One year has about 31,536 seconds. If you divide 3.2 billion by the number of seconds in a year you’ll see it is about 101 years (101.47, to be precise). 3 Fijalkowska, I. J., Schaaper, R. M., and Jonczyk, P. (2012) DNA replication fidelity in Escherichia coli: A multi-DNA polymerase affair. FEMS Microbiology Reviews, vol. 36, issue 6, pp. 1105–1121. DNA Replication M05_NORM8290_01_SE_C05.indd 127 127 24/08/17 10:15 AM Bacterial chromosome The circular chromosome in prokaryotes is folded over on itself in the nucleoid region of the cell. Replication forks 1 Enzymes collect at a specific point on the chromosome and form a bubble with two replication forks at the points of unwinding. Parent strand 2 Within the bubble, new “daughter” strands build on to each original “parent” strand. Replication forks continue advancing at the top and bottom. Daughter strand 3 Eventually the replication forks meet to release two doublestranded circular chromosomes; each chromosome has a new daughter strand and an original parent strand. FIGURE 5.7 DNA replication forks in a circular chromosome 128 TABLE 5.3 Key Enzymes in DNA Replication Enzyme Function Helicase Unwinds DNA helix Primase Builds RNA primers; multiple primers are required to build the lagging strand DNA polymerase III Main enzyme that copies DNA on the leading and lagging strand DNA polymerase I Replaces RNA primers with DNA; also has a role in DNA repair Ligase Forms phosphodiester bonds to seal nicks in the DNA sugar– phosphate backbone; important in DNA replication and DNA repair Gyrase and Topoisomerases Relieve torsion stress that develops ahead of helicase as DNA unwinds As helicase unwinds the DNA, a bubble of single-stranded DNA forms. DNA polymerase III binds DNA within this bubble region and replication starts. The original DNA strands are often called “parent” strands, while the newly made DNA strands are called “daughter” strands. The immediate point where unwinding occurs is called a replication fork. Due to the bidirectional progression of DNA replication, there are two replication forks. As the replication bubble grows, the DNA helix unwinds to the right and left of the origin of replication. Thus, DNA is replicated bidirectionally and proceeds all the way around a circular chromosome until the completed copies separate (FIG. 5.7). At the replication fork, helicase breaks the hydrogen bonds between the nitrogen bases to unzip the DNA strands. Single-strand DNA-binding proteins then bind the DNA strands to keep them separated until they are copied. Specialized detangling enzymes called gyrase and topoisomerases relieve the coiling tension that develops as the helix unwinds. Another enzyme, primase, lays down segments of RNA primers to jump-start replication. DNA polymerase III, the main enzyme that builds DNA, relies on these RNA primers as a springboard to start DNA replication. This is because DNA polymerase III can extend a nucleic acid strand, but it cannot start a new strand from scratch (it requires a 3’ OH group to build upon). FIG. 5.8 shows an overview of DNA replication. Leading and lagging strand replication differs. DNA polymerase III, the primary enzyme that copies DNA, only builds DNA in a 5’ to 3’ direction. This, along with DNA’s antiparallel arrangement, means that one side of the parent DNA molecule is copied in the same direction as helix unwinding—this is called the leading strand. The complementary strand is copied in the opposite direction of unwinding, and is known as the lagging strand. Every replication fork has a leading and a lagging strand. DNA Replication on the Leading Strand On the leading strand, DNA polymerase III launches off of the single RNA primer laid down by primase. The RNA must later be removed, since the completed DNA strand can’t have any RNA in it. As DNA polymerase III advances it lays down new nucleotides that correctly pair with the parent DNA template. If the parent DNA strand has an adenine nucleotide, then the polymerase lays down a complementary thymine nucleotide. Overall, the goal is to make the new DNA strand look exactly like the parent DNA. Because the direction of replication on the leading strand is the same as unwinding, replication is continuous along the template. As mentioned earlier, the RNA primer that was laid down to jump-start replication must be removed before the leading strand is complete. To accomplish this, DNA polymerase I replaces the RNA primer with DNA. The final step is for CHAPTER 5 Genetics M05_NORM8290_01_SE_C05.indd 128 24/08/17 10:15 AM 3ʹ 5ʹ At replication forks 5ʹ 3ʹ 1 Helicase unwinds the DNA. 2 Single-strand binding proteins stabilize single-stranded DNA. 3 Primase lays down one RNA primer on the leading strand and many RNA primers on the lagging strand. 4 DNA polymerase III builds DNA off RNA primers on leading and lagging strand. 5 DNA polymerase I replaces RNA primers with DNA. 6 Ligase glues the lagging strand’s DNA (Okazaki fragments) together at the junctions where RNA primers were removed. Growing daughter DNA strands Okazaki fragments CHEM NOTE Hydrogen bonds hold complementary DNA strands together. These bonds are weaker than covalent and ionic bonds, making it easier to separate the DNA strands for replication. In DNA, three hydrogen bonds form between G-C pairs, while two hydrogen bonds form between A-T pairs. Therefore, the more G-C pairs in a DNA molecule, the harder it is to separate the DNA strands. RNA primer LEADING STRAND 3ʹ 5ʹ LAGGING STRAND 3ʹ 5ʹ FIGURE 5.8 DNA replication on the leading and lagging strands CONCEPT COACH Bring the art to life! Watch the Concept Coach animation and master DNA replication. DNA Replication M05_NORM8290_01_SE_C05.indd 129 129 24/08/17 10:15 AM TRAINING TOMORROW’S HEALTH TEAM Hampering Herpes by Inhibiting DNA Replication Many drugs, from antibiotics to anticancer drugs, work by inhibiting DNA replication. Acyclovir is an example of an Acyclovir drugs limit antiviral drug used the breakout of sores to treat (not cure) outbreaks of genital associated with chickenpox, shingles, and genital herpes. herpes, shingles, cold sores, and chickenpox. Acyclovir is a chemical compound that resembles the guanine nucleotide found in DNA, except it does not have a sugar group, and will therefore cause DNA replication to stop once it is added to the growing DNA strand. Once DNA replication stops, the viral genome cannot be copied, leading to a dead end for the virus, which must copy its genome to make new viral particles. This drug also kills the infected host cell, since it not only blocks viral DNA replication, but it also interferes with host DNA and RNA. Acyclovir is only toxic to cells infected with certain herpes viruses that encode an enzyme that activates acyclovir into a toxic form. Mammalian cells that are not infected with certain herpes viruses will not transform the compound into a toxic form, meaning they can persist in the presence of the drug without harm. Q U EST I ON 5.2 Based on what you have learned about DNA replication, why would adding acyclovir to the growing DNA strand ultimately result in a dead end for DNA replication? the enzyme ligase to glue together the junction between where DNA polymerase I replaced the RNA primer with DNA and the rest of the leading strand. DNA Replication on the Lagging Strand DNA polymerase III builds new DNA on the lagging strand, too. However, since the lagging strand builds in the opposite direction of the helix unwinding, replication is a discontinuous process, where chunks of the lagging strand are constructed in a sort of “back-filling” action. These DNA chunks are made via several enzymes, and are called Okazaki fragments, after the scientist who discovered them. Unlike on the leading strand, where primase synthesizes a single primer, on the lagging strand many RNA primers are laid down at intervals along the DNA template. DNA polymerase III latches onto the end of an RNA primer and builds DNA in a 5’ to 3’ direction, until it encounters the next primer. This results in DNA fragments that are capped at their start by a short RNA sequence. Then DNA polymerase I replaces the RNA primers with DNA. In the final step, ligase glues the multiple Okazaki fragments together, producing an uninterrupted strand. By the end of replication, there will be two separate DNA helices. Each helix contains one original parent DNA strand paired with a newly formed complementary DNA strand. Because DNA replication produces a hybrid molecule that is half new and half parent DNA, it is described as a semiconservative process. Prokaryotes and eukaryotes replicate DNA somewhat differently. Although eukaryotic DNA replication tools differ in structure, number, and sometimes name from what bacteria use, collectively the eukaryotic replication machinery works similarly to that of prokaryotes. That being said, DNA replication in eukaryotes takes longer and involves more protein factors, as eukaryotic genomes tend to be significantly larger and spread across more chromosomes than prokaryotic genomes. An average animal (eukaryotic) cell has about 50 times more DNA to copy than the average bacterial cell. To manage their large genomes, eukaryotic cells depend on higher-order packaging of their DNA, so that the genome can fit into the nucleus. This higher-order organization takes time to undo and then reconstruct, so it slows replication. Also, because eukaryotes have a lot of DNA to copy, they have multiple replication initiation sites on their chromosomes (thousands) versus the single origin of replication seen in most bacteria. However, even the huge number of replication sites doesn’t enable eukaryotic cells to copy their DNA as fast as prokaryotes. Certain bacteria can double their population about every 20 minutes, while eukaryotic cells often take between 18 and 24 hours to complete cell division. BUILD YOUR FOUNDATION Build your foundation by answering the Quick Quiz: scan this code or visit the Mastering Microbiology Study Area to quiz yourself. 130 QUICK QUIZ 8. When do cells carry out DNA replication, why is it essential, and what contributes to its accuracy? 9. How is DNA replication started? 10. What is the main enzyme that builds DNA? Describe at least one of its rules or limitations. 11. What enzyme builds RNA primers, and what role do RNA primers have in DNA replication? 12. How are the leading and lagging strands defined and how is replication performed differently on these strands? 13. What is the role of DNA polymerase I in DNA replication? 14. List three differences in DNA replication in prokaryotes versus eukaryotes. CHAPTER 5 Genetics M05_NORM8290_01_SE_C05.indd 130 24/08/17 10:15 AM BENCH to BEDSIDE Genetically Engineered Bacteria and Medical Therapies The first modern genetic engineering was accomplished in the 1970s, when scientists figured out how to selectively cut and paste sections of DNA to make recombinant gene constructs. E. coli was engineered to produce human insulin (Humulin); in 1983, it became the first recombinant DNA human healthcare product on the market. Since then, bacteria have been actively employed as drug factories for recombinant gene products. Other recombinant DNA products include many antibiotics and vaccines, blood-clotting factors, anti-anemia drugs, drugs to stimulate bone marrow growth following a bone marrow transplant, and various diagnostic tests for HIV, tuberculosis, and several cancers. While the products made by recombinant microbes have been used in medical applications since the early 1980s, the direct use of engineered bacteria in medical therapies is still developing. There has been promising progress in this area, especially in treating inflammatory bowel diseases (IBDs), which include ulcerative colitis and Crohn’s disease. IBD illnesses are characterized by chronic inflammation of the intestines. The administration of anti-inflammatory protein, interleukin-10 (IL-10), has been shown to alleviate the inflammation in IBD. Barriers to using IL-10 in therapy are that stomach acid destroys it before it reaches the intestines and injections are poorly tolerated. Engineered bacteria are a possible alternative to deliver IL-10 treatments. Lactococci, which naturally live in acidic environments, can survive the stomach and temporarily grow in the bowel, making them ideal for delivering compounds such as IL-10 directly to the intestines. Plus, these bacteria are safe to consume; they are abundant in dairy products like yogurt and cheeses. Seizing on this collection of knowledge, scientists have engineered Lactococcus lactis to secrete human IL-10. Results suggest that these IL-10 engineered bacteria are effective at treating irritable bowel disease (IBD) in tested mouse models and they have been effective in phase I clinical trials. Sources: Steidler, L., Hans, W., Schotte, L., Neirynck, S., et al. (2000) Treatment of murine colitis by Lactococcus lactis secreting interleukin-10. Science, vol. 289, pp.1352–1355. Braat, H., Rottiers, P., Hommes, D. W., et al. (2006) A phase I trial with transgenic bacteria expressing interleukin-10 in Crohn’s disease. Clinical Gastroenterology and Hepatology, vol. 4, no. 6, pp. 754–759. Del Carmen, S., De Moreno de LeBlanc, A., Perdigon, G., et al. (2012) Evaluation of the anti-inflammatory effect of milk fermented by a strain of IL-10-producing Lactococcus lactis using a murine model of Crohn’s disease. Journal of Molecular Microbiology and Biotechnology, vol. 21(3–4), pp. 138–146. PROTEIN SYNTHESIS (GENE EXPRESSION) Protein synthesis entails reading the genomic instruction manual using transcription and translation. In the last section you learned how cells copy their entire instruction manual and transfer it to a new generation of cells using DNA replication. Here we discuss protein synthesis, also known as gene expression, where genetic information within a cell is read and used to create gene products—proteins. Unlike DNA replication that copied the whole genome, in protein synthesis only parts of the genome are read at any given time. What is read (or what genes are expressed) depends on the cell’s circumstances. As an analogy, you may have access to a full library of information, but you won’t read a book on Shakespeare if your task is to write a paper on cells. Similarly, cells read the parts of their genetic instruction manual that will help them with a necessary task. Protein synthesis is central to life, as it is responsible for most physical and functional cell features. Making proteins is so important that if it is blocked the cell will eventually die. Many antibiotics work by blocking protein synthesis. Protein synthesis has two main stages: transcription and translation. In transcription genes in DNA are copied into a new format, RNA. The cell’s DNA is not changed in this process. The second step, translation, entails reading mRNA to build proteins. After reading this section, you should be able to: 13 Explain what protein synthesis (gene expression) is and describe how it impacts phenotype. 14 Explain the steps of protein synthesis and point out differences in the process in prokaryotes versus eukaryotes. 15 Describe structures and functions of the three RNA types. 16 Discuss the features of the genetic code and how these features benefit a cell. 17 Define reverse transcription. 18 Summarize mRNA splicing in eukaryotes. 19 Explain what nonstandard genetically encoded amino acids are and how they can be incorporated into proteins. 20 Define and give examples of post-translational modifications. Transcription makes RNA. Transcription makes RNA in three main steps: (1) initiation, (2) elongation, and (3) termination. RNA polymerase performs transcription (FIG. 5.9). (1) In initiation RNA polymerase binds to the promoter—the beginning region of a gene in DNA. Once bound to the promoter, RNA polymerase unwinds the DNA to reveal a single-stranded template of the gene that will Protein Synthesis (Gene Expression) M05_NORM8290_01_SE_C05.indd 131 131 24/08/17 10:15 AM TRANSCRIPTION TRANSLATION Summary Step details 3΄ 5΄ First stage in protein synthesis: Occurs in three steps: initiation, elongation, and termination. Uses DNA as a template to make all RNAs in cell, including mRNA, tRNA, and rRNA. 1 Initiation RNA polymerase RNA polymerase binds to the promoter and DNA unwinds, revealing the template strand. DNA Promoter sequence Main enzyme is RNA polymerase. 2 Occurs in cytoplasm in prokaryotes. Occurs in nucleus in eukaryotes. Uses a lot of cellular energy. Tightly regulated by cells. 3΄ 5΄ 5΄ Template strand Elongation RNA polymerase travels down the DNA. The RNA is built from 5΄ to 3΄ as complementary ribonucleotides are paired with the template strand. 3΄ DNA template strand A U T A G C C G RNA G 3΄ 3΄ A U C G Completed new RNA 3 Termination 5΄ 3΄ 3΄ DNA template strand Resulting RNA strand 5΄ Ribonucleotides G 5΄ Growing RNA 5΄ Growing T Transcription 5΄ A termination sequence at the end of the transcribed gene signals the RNA polymerase to fall off the DNA and release the new RNA. Termination sequence 3΄ 5΄ 3΄ FIGURE 5.9 Transcription Transcription is the first stage of protein synthesis. Critical Thinking Transcription generates a different version of the same information. Explain this statement. be transcribed into RNA. (2) During elongation, the enzyme advances along the single-stranded DNA, laying down complementary ribonucleotides as it goes. (Recall that in RNA U pairs with A, and G pairs with C.) (3) The termination step occurs when the RNA polymerase reaches the end of the gene. At this point, a termination sequence signals the polymerase to fall off the DNA, and the newly made RNA transcript is released. Transcription occurs in the nucleus in eukaryotes and in the cytoplasm of prokaryotes. Reverse Transcription While genetic information usually flows just one way (from DNA to RNA to proteins), some cells and viruses can carry out reverse transcription, which uses RNA as a template to build copy DNA (cDNA). Special enzymes called reverse transcriptases carry out reverse transcription. Certain viruses (including retroviruses such as HIV) and certain human cells make reverse transcriptase enzymes. 132 CHAPTER 5 Genetics M05_NORM8290_01_SE_C05.indd 132 24/08/17 10:15 AM Three main types of RNA are involved in protein synthesis. Using DNA as the guiding instructions, transcription makes all the RNA molecules in a cell. (FIG. 5.10). Cells make numerous types of RNA, but there are three main types of RNA involved in protein synthesis. 1. Messenger RNA (mRNA): As their name indicates, these mostly linear molecules carry the genetic messages stored in DNA. Messenger RNA is encoded in triplets of nucleotides called codons. Codons are only found in mRNA. They consist of A, U, G, and C, and each codon specifies either an amino acid, or a stop signal that ends translation. In prokaryotes, one mRNA molecule can carry a code for several different proteins and is said to be polycistronic. In eukaryotes, mRNA is often (although not always) monocistronic, or codes for one protein.4 2. Transfer RNA (tRNA): These cloverleaf-shaped RNA molecules bring the correct amino acid to a ribosome to build proteins. An amino acid is bonded to the 3’ end of the molecule to be shuttled to a ribosome. The anticodon loop, located toward the lower portion of the tRNA, is complementary to a codon on mRNA. This ensures that the proper amino acid is being brought into the ribosome to be added to the growing protein chain. RNA splicing involves clipping out certain sequences in RNA and joining the remaining parts of the molecule. Splicing occurs in many types of RNA in prokaryotes and eukaryotes, but here we focus on mRNA splicing in eukaryotes. In eukaryotic cells only certain segments of mRNA are decoded to build a protein—these coding sequences are called exons. The intervening sequences in mRNA that are not decoded to build the protein are called introns. Even though intron sequences aren’t decoded to contribute to the protein, they are still important tools in protein synthesis. Examples of intron function include regulating how much of a protein is made and determining what versions of the protein are present in a cell. While introns were originally thought to be strictly in eukaryotes, they have been found in prokaryotic RNAs5––although not in prokaryotic mRNA. Consequently, prokaryotic mRNA does not need to be spliced before it is translated into protein. However, in eukaryotic cells, mRNA has to be spliced and transported out of the nucleus before it can be translated. Eukaryotic cells remove the introns in their mRNAs, and join the exons so that a coherent message is decoded by the ribosome. This mRNA editing is performed in the nucleus by Messenger RNA (mRNA) carries a genetic message in triplet code (codons) and is translated to build a protein. 5´ Amino acid 5´ 3´ Transfer RNA (tRNA) This cloverleaf shaped molecule serves as an adaptor molecule to usher amino acids into the ribosome during translation. GCU 3. Ribosomal RNA (rRNA): These RNA molecules fold up into elaborate threedimensional structures and combine with proteins to form ribosomes. There are several types of rRNA in ribosomes and there is impressive diversity regarding rRNA sequences among species. This makes rRNA sequencing a useful tool for taxonomically grouping organisms. Despite the diversity of rRNA sequences, the general functions of rRNAs are the same; they all help ribosomes “read” or scan an mRNA molecule and form peptide bonds between amino acids to build a protein. Splicing mRNA 3´ Codon Anticodon Ribosomal RNA (rRNA) rRNA Proteins takes on complex stem and loop structures and combines with proteins to build ribosomes. FIGURE 5.10 RNAs Three classes of RNA participate in translation. 4 The existence of polycistronic messages in eukaryotes is a fairly recent concept and is reviewed in Blumenthal, T. (1998) Gene clusters and polycistronic transcription in eukaryotes. Bioessays, vol. 20, pp. 480–487. 5 Four main classes of introns have been identified in nature. Prokaryotic introns fall into “selfsplicing” classes and do not require the spliceosome machinery seen in eukaryotes. Protein Synthesis (Gene Expression) M05_NORM8290_01_SE_C05.indd 133 133 24/08/17 10:15 AM a complex called the spliceosome (FIG. 5.11). The advantage of mRNA splicing is that there is the potential for alternative splicing, or new combinations of exons. Therefore, introns shouldn’t be perceived as junk or a nuisance; rather, they provide an opportunity for genetic diversity. Ribosomes translate mRNA to build proteins. The ribosome serves as an efficient translation machine. Recall (from Chapters 3 and 4) that ribosomes have a large and a small subunit. These subunits combine to make an active ribosome that performs translation. To understand how translation occurs, it is useful to think about written language. Letters form words; words build sentences; and punctuation indicates the beginning and end of each sentence. A cell’s nucleotides can be thought of as its alphabet. These letters are organized into three-letter words—the codons in mRNA. In addition to specifying words, some codons act as punctuation, telling the cell where to begin or end a sentence. Just as we read words and punctuation and extract the meaning from a sentence, cells read transcripts and use them to assemble the specified protein. Because there are only four letters in a cell’s alphabet (A, U, G, and C), and all of a cell’s words are three letters long, there are only 64 possible words in a cell’s vocabulary (4 * 4 * 4 = 64). These 64 codons encode for 22 amino acids, three stop signals, and one start signal. Codons that encode amino acids are called sense codons, while those that encode stop signals are called nonsense codons. The triplet code encoded by the codons of mRNA directs the production of all proteins. Model showing 3D structure of tRNA The Genetic Code and Amino Acids In nature there are hundreds of amino acids, but as already mentioned, to date only 22 are known to be genetically encoded by codons. Of these, 20 are called standard amino acids, while the other two (selenocysteine and pyrrolysine) are considered nonstandard for reasons that we will discuss later. Standard amino acids are typically depicted in genetic code tables like that in TABLE 5.4. Looking TABLE 5.4 The Genetic Code Second Base First Base U U UUU UUC UUA UUG C A G 134 Phenylalanine Leucine CUU CUC CUA CUG Leucine AUU AUC AUA Isoleucine AUG Methionine GUU GUC GUA GUG Valine C A UCU UCC UCA UCG UAU UAC CCU CCC CCA CCG ACU ACC ACA ACG GCU GCC GCA GCG Serine Proline Threonine Alanine G Tyrosine UGU UGC UAA UGA UAG UGG CAU CAC CAA CAG Histidine Glutamine Third Base Cysteine U C A Tryptophan G CGU CGC CGA CGG Arginine U C A G AAU AAC Asparagine AGU AGC Serine U C AAA AAG Lysine AGA AGG Arginine A G GAU GAC Aspartate GGU GGC Glutamate GGA GGG GAA GAG Glycine U C A G CHAPTER 5 Genetics M05_NORM8290_01_SE_C05.indd 134 24/08/17 10:16 AM EUKARYOTIC CELL PROKARYOTIC CELL In nucleus: In cytoplasm: TRANSCRIPTION TRANSCRIPTION mRNA PROCESSING In cytoplasm: TRANSLATION TRANSLATION Eukaryotic cells must process their mRNA before translation 1 Eukaryotic mRNA contains non-proteinencoding introns. Intron Exon 1 Exon 2 Exon 3 Spliceosome 2 bycreates spliceosome a coherent Intron removal protein-encoding mRNA strand. mRNA 3 Processed with joined exons is ready to be exported to the cytoplasm to be translated. Exons 1–3 joined together Exon 1 Exon 2 Exon 3 FIGURE 5.11 Eukaryotic mRNA processing The spliceosome removes introns and join exons. Critical Thinking How could an alternative protein be made from the portrayed mRNA? at the table, you will notice that a single amino acid can be represented by more than one codon (for example, there are six codons for leucine). This redundancy in the genetic code protects cells from genetic changes (mutations). In redundant codons the first two nucleotides tend to remain constant, while the third nucleotide, sometimes referred to as the wobble position, differs. Overall, translation is a rapid and accurate process. Under optimal conditions, E. coli can add up to 20 amino acids per second to a growing protein chain and only misreads about one in 10,000 codons. CHEM NOTE Amino acids are the building block of proteins. They have a core structure consisting of an amine group (NH2) and a carboxyl group (COOH). Protein Synthesis (Gene Expression) M05_NORM8290_01_SE_C05.indd 135 135 24/08/17 10:16 AM Step 1 of Translation: Initiation Bring the art to life! Watch the Concept Coach animation and master Protein Synthesis. CONCEPT COACH Earlier you learned that transcription builds RNA in three steps, initiation, elongation, and termination. Here you’ll see those same three stepwise terms again. However, this time they describe the steps in translation––the process that ribosomes use to build proteins. The ribosome serves as an efficient translation machine. Recall that ribosomes have two subunits, a large and a small subunit. These subunits combine to make an active ribosome, which has an exit site (E), a peptidyl site (P), and an acceptor site (A). These sites coordinate translation (FIG. 5.12). During initiation, a ribosome attaches to the mRNA and scans it until it encounters a start codon, where it adds the first amino acid. Several protein factors help initiation in prokaryotes, while over a dozen factors help initiation in eukaryotes, but we will discuss the process in simplified terms. Usually the start codon is AUG, but no matter what is designated as the start codon, the first amino acid added by the initiator tRNA in prokaryotes is formyl methionine (fMet). In eukaryotes, the first amino acid added is simply methionine (Met). While the beginning amino acids don’t vary during translation, these amino acids may be clipped off later, when the protein is finalized. In either case, the initiator tRNA enters the ribosome at the P site to start translation. In prokaryotes, ribosomes can bind to mRNA and start translating it into protein even before transcription concludes. This “hot off the presses” translation is possible because prokaryotes do not have to splice their mRNA before translating it, and their mRNA does not have to be shipped out of a nucleus. In eukaryotic cells, both RNA splicing and nuclear export must occur before translation begins. Regardless of the type of cell involved, once translation begins, it is possible for multiple ribosomes to latch onto a single mRNA molecule. These socalled polysomes, or collections of many ribosomes on a single mRNA, allow prokaryotes and eukaryotes to rapidly produce proteins from mRNA transcripts (FIG. 5.13). Step 2 of Translation: Elongation CHEM NOTE Peptide bonds are a type of covalent bond in which the amine group of one amino acid covalently bonds with the carboxyl group of an adjacent amino acid. Peptide bonds link amino acids together to form polypeptides, or proteins. This chemistry happens between amino acids in the P and A sites of the ribosome. During elongation, the ribosome constructs or elongates the protein. Once ribosomes attach to the mRNA, transfer RNAs (tRNAs) shuttle amino acids to them. Each genetically encoded amino acid has at least one specific tRNA molecule. Because there are 22 genetically encoded amino acids, there are at least 22 different tRNAs. A tRNA that is carrying its specific amino acid is said to be “charged.” One at a time, charged tRNAs enter the ribosome at the A site and try to pair with a codon on the mRNA. If the tRNA’s anticodon loop is complementary to the mRNA codon (by base-pairing rules), then the amino acid is added to the growing protein chain. The codon/anticodon pairing process ensures that the manufactured protein follows the genetic instructions in mRNA. Assuming the mRNA’s codon and the tRNA’s anticodon are complementary, the ribosome forms a peptide bond between adjacent amino acids. The ribosome then shifts along the mRNA, kicking the uncharged tRNA out of the ribosome (via the E site) to make room for the next charged tRNA to enter the ribosome. Step 3 of Translation: Termination A ribosome continues the elongation process, adding amino acids to the growing protein chain at each codon on the mRNA, until it reaches a stop codon (or nonsense codon) that signals termination––the end of translation. Three codons serve as termination or stop signals (see Table 5.4) that encourage termination factors to enter the ribosome, end translation, and release the completed protein. Eventually the ribosome detaches from the mRNA, enabling it to then reattach to the start codon of the same (or another) mRNA and begin the translation process anew. 136 CHAPTER 5 Genetics M05_NORM8290_01_SE_C05.indd 136 24/08/17 10:16 AM TRANSLATION TRANSCRIPTION Summary Step details Second stage in protein synthesis: 1 Occurs in three steps: initiation, elongation, and termination. With the help of tRNA, ribosomes decode/read mRNA to make a protein. Start codon Initiation Ribosome attaches to mRNA and scans until it reaches a start codon (usually AUG). mRNA A U G E Initiator tRNA carrying amino acid methionine then enters the ribosome’s P site. P Process occurs in the cytoplasm in both prokaryotes and eukaryotes. Initiator tRNA Uses a lot of cellular energy. Tightly regulated by cells. 5´ A 2 Elongation Key players 2d E E site (exit site) P P site (peptidyl site) A A site (acceptor site) Cycle repeats until stop codon encountered. Ribosome Amino acid 2c 2a Ribosome translocates (shifts) down mRNA: tRNA in P site shifts to E site to exit ribosome; tRNA in the A site shifts to P site. Incoming tRNA enters A site. 2b Peptide bond forms between amino acids transferring growing protein to tRNA in A site. Anticodon tRNA Codon mRNA G A A C U U 3 Termination Ribosome encounters stop codon. Termination factor enters the ribosome. Anticodon/ codon pair Ribosome releases the protein and detaches from mRNA. U Termination factor G A Released protein Stop codon 3´ FIGURE 5.12 Translation overview Critical Thinking Describe two possible ways that tRNA could introduce a mutation in translation. Protein Synthesis (Gene Expression) M05_NORM8290_01_SE_C05.indd 137 137 24/08/17 10:16 AM In eukaryotes, most mRNA molecules can only encode a single protein. But this is not the case in prokaryotes, whose mRNAs are commonly polycis3ʹ tronic, meaning they can encode multiple types of proteins. Thus, in prokaryotes the ribosome may not immediately detach from the mRNA when it encounters a stop codon. Instead, part of the ribosome often remains on the mRNA mRNA and continues reading it in search 5ʹ RNA of the next start codon. Having ribosomes polymerase DNA (pink) Multiple ribosomes 3ʹ remain on the mRNA to check for additional start codons is very efficient for Direction of RNA prokaryotic cells. Direction each of the polymerase movement For many years it was thought that three pictured ribosomes for transcription move for translation stop codons always terminated translation. The discovery of the nonstandard genetically encoded amino acids changed mRNA (yellow) this thinking. Under specific circumstances, the stop codon UGA encodes a nonstandard amino acid called selenocysteine. Initially it was thought that UGA’s Ribosomes (teal) ability to code this was unique to bacteria 5ʹ TEM showing simultaneous transcription and archaea, but we now know that this and translation. also occurs in many eukaryotes, includDNA ing humans. Mutations or deficiencies in FIGURE 5.13 Polysome formation In bacteria, polyribosomes (or polysomes) can develop before proteins that contain selenocysteine have transcription finishes. In eukaryotes (not shown), the mRNA has to be processed and exported out been associated with cancer, male inferof the nucleus before translation can start. However, once the mRNA is in the cytoplasm, multiple tility, Keshan disease (a heart condition), ribosomes can translate it in parallel. thyroid hormone abnormalities, and diminished immune response. Similarly, the nonstandard amino acid pyrrolysine can be incorporated into proteins under specific circumstances by the stop codon UAG. So far, only certain prokaryotes (mainly methanogenic archaea) seem to use pyrrolysine. Translation termination does not necessarily mark the end of the protein’s development. Once released from the ribosome, proteins often undergo modifications such as trimming; they may also have various organic factors (e.g., sugars and lipids) and inorganic factors (e.g., phosphate and metal ions) added to them. These post-translational modifications are often required for a protein to function and provide a way for cells to regulate gene product functionality. Growing protein BUILD YOUR FOUNDATION Build your foundation by answering the Quick Quiz: scan this code or visit the Mastering Microbiology Study Area to quiz yourself. 138 QUICK QUIZ 15. If a cell could not express genes, what would be the ultimate consequence, and why? 16. What is transcription, and what enzyme is primarily responsible for it? 17. Name and describe three different types of RNA that are made by transcription. 18. What is the general function of reverse transcriptase? 19. What are introns, and how do they impact protein synthesis? 20. Explain the term “redundancy” as it relates to the genetic code. 21. What is meant by “triplet code”? 22. Compare and contrast translation in prokaryotes versus eukaryotes. 23. What is an anticodon, where is it found, and how does it factor into protein synthesis? 24. Name two nonstandard genetically encoded amino acids and describe how they are incorporated into a protein. 25. Why are post-translational modifications important? CHAPTER 5 Genetics M05_NORM8290_01_SE_C05.indd 138 24/08/17 10:16 AM REGULATING PROTEIN SYNTHESIS Controlling protein synthesis is essential for all cells. Protein synthesis through transcription and translation is energy and resource intensive, so it is essential for cells to control when these processes occur. As mentioned previously, cells do not constantly express every gene in their genome. Typically less than 20 percent of a cell’s genes are expressed at any given time. Some are used for everyday cell work and are referred to as housekeeping genes—these genes encode proteins that are reliably found in a cell, and are thus considered constitutive genes. In contrast, proteins from facultative genes are made selectively, when a cell encounters a specific environmental change, or has a special job to do. Of E. coli’s approximately 4,200 proteinencoding genes, only about 250 are considered constitutive.6 Even when a gene is switched on, the amount of a gene product made is still carefully regulated. Protein synthesis is usually regulated at the pre-transcription and posttranslation levels (FIG. 5.14). After reading this section, you should be able to: 21 Describe the difference between constitutive and facultative genes. 22 Summarize the general effect of pretranscriptional regulation and the basic role of transcription factors in regulation. 23 Define the parts of an operon, and compare and contrast inducible and repressible operons. 24 Explain what epigenetic regulation is, and how it can impact protein synthesis. 25 Describe the role of quorum sensing in protein synthesis, and how this enhances bacterial survival. 26 Define post-transcriptional regulation and provide specific examples of it. Eukaryotic cell Prokaryotic cell TRANSCRIPTION TRANSLATION IN BOTH EUKARYOTES AND PROKARYOTES Pre-transcriptional regulation allows cell to control gene expression by regulating how much mRNA is made from a given gene. Post-transcriptional regulation allows cell to control gene expression by regulating how often mRNA is translated into protein. Examples: Examples: Operons Quorum sensing Epigenetic control (such as DNA methylation) Recruitment of transcription factors Recruiting ribosomes to mRNA Controlling mRNA stability Small noncoding RNAs Riboswitches Eukaryotes also can control RNA processing and nuclear export. (This is not used by prokaryotes.) FIGURE 5.14 Regulating protein synthesis by controlling transcription and/or translation Cells regulate gene expression at a variety of levels. Two key points of regulation are at the pre-transcriptional and post-transcriptional levels. Critical Thinking Why could pre-transcriptional regulation be considered more energy efficient than posttranscriptional regulation? 6 Puigb, P., Romeu, A., and Garcia-Vallv, S. (2008) HEG-DB: A database of predicted highly expressed genes in prokaryotic complete genomes under translational selection. Nucleic Acids Research, vol. 36, pp. D524–D527. Regulating Protein Synthesis M05_NORM8290_01_SE_C05.indd 139 139 24/08/17 10:16 AM The Case of the Spreading Superbug CLINICAL CASE NCLEX HESI TEAS Practice applying what you know clinically: scan this code or visit the Mastering Microbiology Study Area to watch Part 2 and practice for future exams. Pre-transcriptional regulation impacts when and how often transcription occurs. Pre-transcriptional regulation helps cells control when and how often transcription occurs (regulates RNA production). It is a tremendously important mechanism for controlling protein synthesis. There are a variety of transcriptional regulation mechanisms, but all affect how much RNA is made from a given gene. Most forms of pre-transcriptional regulation are managed by transcription factors––specialized proteins that bind to DNA and help recruit RNA polymerase to start transcription. By controlling the presence and action of transcription factors, cells exert amazing control over protein synthesis. Bacterial cells experience frequent and dramatic changes in their environment. To survive, they must be ready to rapidly respond to such changes. As such, bacteria are excellent models for understanding how protein synthesis is regulated. Next we will examine operons, one important type of pretranscriptional regulation of protein synthesis. Operons are one form of pre-transcriptional regulation. Operons are a collection of genes controlled by a shared regulatory element. The genes in an operon participate in a joint goal, such as building a specific substance, or breaking something down. Think of operons as an efficient way to coordinate an assortment of sports team members who must work together to make a winning play. It was originally thought that only prokaryotic cells used operons, but operons have also been found in certain eukaryotes.7 Operons include four key parts that combine to make an on-off switch to regulate transcription. These four parts are (1) a promoter, which RNA polymerase associates with to start transcription; (2) two or more genes that encode proteins that work together toward a shared task; (3) a repressor that blocks transcription; and (4) an operator, the part of the operon that the repressor binds to in order to block transcription. When the repressor binds the operator, transcription is blocked and the operon is “off.” When it falls off the operator, the genes in the operon are transcribed and the operon is “on.” There are two main classes of operons. Inducible operons are off by default unless certain conditions arise under which they are activated (induced) to allow transcription. In contrast, repressible operons are on by default, meaning they are actively transcribed until they are switched off (repressed). As an analogy for inducible operons, think of a car’s headlights, which are by default off unless you are induced to turn them on by dark conditions. Repressible operons can be thought of as akin to the electricity supply to your home, which is always on and ready to power devices or appliances, unless a power outage results in the power being shut off, or repressed. Inducible Operons An example of an inducible operon is the lactose (lac) operon of E. coli. There are three structural genes in the lac operon that help the cell digest lactose. This operon is induced, or actively transcribed, only when lactose is present and the cell’s preferred food, glucose, is absent (FIG. 5.15). Unless both conditions are met, the repressor remains attached to the operator, blocking transcription. When lactose is present and glucose is absent, the cell makes an inducer called allolactose, which inactivates the repressor and prevents it from binding 7 Blumenthal, T. (2004) Operons in eukaryotes. Briefings in Functional Genomics, vol. 3, pp. 199–211. Lawrence, J. G. (2002) Shared strategies in gene organization among prokaryotes and eukaryotes. Cell, vol. 110, pp. 407–413. 140 CHAPTER 5 Genetics M05_NORM8290_01_SE_C05.indd 140 24/08/17 10:16 AM Operon OFF Lactose absent and glucose present Operon ON Lactose present and glucose absent DNA Repressor 1 2 3 4 Active repressor made. Repressor Regulatory gene 2 Allolactose not present, so repressor remains active. Repressor binds operator and blocks RNA polymerase. 1 RNA polymerase (blocked by repressor) RNA polymerase can’t transcribe the structural genes, so lactose-utilization proteins are not made. Active repressor made. Inducer allolactose made when lactose is present and glucose is absent. Allolactose RNA polymerase Promoter Operator Lactoseutilization genes 3 4 Inducer inactivates repressor so it can’t bind the operator; RNA polymerase is no longer blocked. Lactoseutilization proteins RNA polymerase transcribes structural genes in the operon and the proteins to utilize lactose are made. mRNA Lactoseutilization genes transcribed Translation DEFAULT STATE to the operator. With the repressor removed, RNA polymerase’s path is no longer blocked and it can transcribe the structural genes in the operon. If all the lactose is digested, or if glucose becomes available again, the cell will stop making allolactose, and the repressor will reassociate with the operator, once again blocking transcription. FIGURE 5.15 Inducible lac operon By default the repressor is active. Unless the repressor is inactivated, the operon is OFF. Repressible Operons Repressible operons allow cells to halt production of substances that are already abundant. One common example is the arginine (arg) operon, which regulates the production of the amino acid arginine. Of course, the cell doesn’t need to make arginine if there is already plenty of it available. The arg operon is by default transcribed (is always “on”) unless the repressor gains the ability to bind to the operator (FIG. 5.16). The repressor can only associate with the operator when it is bound to arginine. Therefore, when arginine is present in sufficient quantities, it will enable the repressor to bind to the operator to block transcription. When the supply of arginine diminishes to a level at which the cell needs more, the repressor dissociates from arginine and falls off of the operator, allowing transcription to resume. Regulating Protein Synthesis M05_NORM8290_01_SE_C05.indd 141 141 24/08/17 10:16 AM Operon ON Operon Cell is low on arginine OFF Cell has plenty of arginine DNA Inactive repressor 1 Inactive repressor Regulatory gene Inactive repressor made. 1 Inactive repressor made. Arginine 2 Arginine Arginine is not present in sufficient quantities to activate the repressor. Promoter 2 Abundant arginine activates repressor. 3 Activated repressor binds operator and blocks RNA polymerase. 4 RNA polymerase can’t transcribe the structural genes, so proteins to build arginine are not made. RNA polymerase 3 4 Repressor can’t bind to operator because it’s not associated with arginine. Arginine-building proteins RNA polymerase mRNA transcribes the structural genes and proteins to build Translation arginine are made. Operator Argininebuilding genes transcribed RNA polymerase DEFAULT STATE FIGURE 5.16 Repressible arg operon By default the repressor is inactive. Unless the repressor is activated by arginine, the operon is ON. Cells alter their epigenome as a means of pre-transcriptional regulation. We have already learned that a genome is all of the genetic material in a cell. An epigenome, by contrast, is the collection of all the chemical changes to the genome. Epigenetic regulation is a way to control protein synthesis by directly altering the appearance of DNA without changing its sequence. One way eukaryotic and prokaryotic cells do this is by DNA methylation, which involves adding methyl groups (CH3 groups) to DNA. Methylating a gene silences it by preventing transcription (FIG. 5.17). DNA methylation patterns have important roles in the development of different tissues in multicellular organisms. This is because a cell’s phenotype is based not only on the genes that were inherited, but also on which genes are expressed. All of the cells in a multicellular organism have the same genome, yet they can be structurally and functionally diverse. How can skin or liver cells be so different in appearance and function, yet both have the same genome? As it turns out, DNA methylation patterns “teach” cells how to develop and function by affecting protein synthesis. DNA methylation patterns can also be inherited, or passed from one cell generation to the next; this heritability of methylation is thought to be just as important to the function of cells as inheritance of the genome itself. 142 CHAPTER 5 Genetics M05_NORM8290_01_SE_C05.indd 142 24/08/17 10:16 AM Although the particulars of DNA methylation are not completely understood, research has shown that when methylation patterns are changed, there are dramatic affects. Certain cancer cells have altered DNA methylation patterns compared with noncancerous cells in the same tissue.8 Also, many agents that cause birth defects have been shown to alter DNA methylation patterns. In bacteria, DNA methylation patterns have diverse roles, from coordinating DNA repair and responses to environmental stress to affecting a bacterium’s ability to cause disease.9 Methylation patterns of bacterial genomic DNA also protect bacteria from infection by bacteriophages, which are viruses that infect bacteria (bacteriophages are discussed further in Chapter 6). Many bacteria use quorum sensing to pre-transcriptionally control protein synthesis. Although bacteria are unicellular organisms, they often exist in communities where they communicate using chemical messengers called autoinducers. These molecules allow bacteria to sense what is occurring in their community and respond accordingly to enhance their chance of survival. The collective sensing and responding to changes within a bacterial community is called quorum sensing. Quorum sensing allows bacteria to alter their protein synthesis in response to changes in the density of the population. In a way, quorum sensing allows unicellular organisms to coordinate their processes and behave more collectively, almost like a primitive multicellular organism. Coordinated protein synthesis through quorum sensing is the foundation for forming biofilms. (See Chapter 1 for more on biofilms.) C G G C A T T A C G G C G C A T T A C G C G Methyl groups are added to cytosine (C) at selected sites. FIGURE 5.17 DNA methylation Adding methyl groups (CH3) to selected cytosine in DNA silences genes by blocking transcription. Post-transcriptional regulation impacts how often mRNA is translated into protein. In contrast to all the previously mentioned pre-transcriptional regulation methods, which act before mRNA is even made, post-transcriptional regulation impacts how often mRNA is translated into protein. Cells can regulate translation by affecting how readily ribosomes associate with mRNA to read it. Cells may also alter mRNA stability; mRNA with a fleeting existence has a limited amount of time to be translated, while stable mRNA exists longer in the cell, providing more opportunities for translation. As previously mentioned, in eukaryotes mRNA is spliced and exported out of the nucleus before translation occurs. Regulating the rate of these processes is another way for eukaryotes to regulate translation. Small Noncoding RNAs In addition to the various RNAs you have already learned about, cells produce a variety of small noncoding RNAs that impact protein synthesis. In eukaryotic cells, small noncoding RNAs that work in post-transcriptional regulation include microRNAs and short interfering RNAs. In prokaryotes there are similaracting RNAs called small RNAs. These regulatory RNAs limit protein synthesis by binding to mRNAs using complementary base-pairing, thereby decreasing the rate of mRNA translation. When these small RNAs associate with mRNA, the mRNA is usually either tagged for destruction, or struggles to have effective interactions with ribosomes. Either way, this reduces how much protein is made from a particular mRNA. In eukaryotic cells, small noncoding RNAs 8 Reviewed in Pollock, R. M. and Richon, V. M. (2009) Epigenetic approaches to cancer therapy. Drug Discovery Today: Therapeutic Strategies, vol. 6, issue 2, pp. 71–79. 9 Casadesus, J. and Low, D. (2006) Epigenetic gene regulation in the bacterial world. Microbiology and Molecular Biology Reviews, vol. 70, pp. 830–856. Regulating Protein Synthesis M05_NORM8290_01_SE_C05.indd 143 143 24/08/17 10:16 AM can also reduce the rate of mRNA processing and nuclear export, ultimately decreasing translation of the affected mRNA. The world of small noncoding RNAs is an active area of research, and could give us further insight into certain mechanisms of bacterial and viral pathogenesis, as well as the progression of various cancers and inherited disorders. Riboswitches Certain mRNAs have built-in switches that act as protein synthesis controls. These riboswitches are not translated into protein. Riboswitches are common gene regulation tools in many bacteria. For example, it is estimated that about 2 percent of the genes in the bacterium Bacillus subtilis are regulated by riboswitches.10 Similar RNA-based switches have been discovered in certain archaea and eukaryotes, too. Diverse riboswitches detect and respond to a wide variety of signals. As a whole, they act as effective tools for regulating transcription at the pre-transcriptional and/or post-transcriptional level. BUILD YOUR FOUNDATION Build your foundation by answering the Quick Quiz: scan this code or visit the Mastering Microbiology Study Area to quiz yourself. 26. 27. 28. 29. 30. 31. 32. 33. QUICK QUIZ How are facultative genes different from constitutive genes? Compare and contrast inducible and repressible operons. Why are transcription factors important regulators of pre-transcriptional control? What is DNA methylation, and how can it impact protein synthesis? How is quorum sensing accomplished, and what effect does it have on bacteria? What is the general goal of post-transcriptional regulation? Explain what small noncoding RNAs are, and how they affect protein synthesis. What are riboswitches, and how can they impact protein synthesis? 10 Mandal, M., Boese, B., Barrick, J. E., Winkler, W. C., and Breaker, R. R. (2003) Riboswitches control fundamental biochemical pathways in Bacillus subtilis and other bacteria. Cell, vol. 113, pp. 577–586. MUTATIONS After reading this section, you should be able to: 27 Describe how mutations contribute to evolution. 28 List and describe the three main categories of mutations. 29 Compare silent, missense, and nonsense mutations. 30 Describe what frameshift and deletion mutations are, and how their impact can be minimized. 31 Explain what a spontaneous mutation is and discuss the primary contributors to spontaneous mutations. 32 Discuss the various classes of mutagens and how they can induce mutations. 33 Summarize what the Ames test is, how it works, and why it is useful. 34 Explain what excision repair is, and how it protects cells from mutations. There are three main categories of mutations: substitutions, insertions, and deletions. A mutation is a change in the genetic material of a cell or virus. Mutations are essential to evolution and variation within species. In single-celled organisms a mutation is more likely to be passed to daughter cells, but in a multicellular organism most mutations are not inherited by offspring. This is because in multicellular organisms the mutation would have to be in a germ line cell (a sex cell like egg and sperm) as opposed to a somatic cell (body cell, such as liver or skin). This is just one reason why evolution rates tend to be faster in unicellular organisms than in multicellular organisms. The three main classes of mutations are substitutions, insertions, and deletions. 1. Substitution mutations occur when an incorrect nucleotide is added—for example, adding a nucleotide that contains guanine instead of thymine. Cells may make this sort of error when replicating or repairing DNA. Sometimes these are called point mutations, because they occur at a specific point, affecting a single base at a time. 2. Insertion mutations occur when a cell adds one or more nucleotides to its genome sequence. 3. Deletion mutations occur when one or more nucleotides are removed from a genome sequence. 144 CHAPTER 5 Genetics M05_NORM8290_01_SE_C05.indd 144 24/08/17 10:16 AM The effect of a base substitution on a cell’s phenotype depends on what substitution is made and where it occurs. The impact of insertions and deletions on the cell’s phonotype depends on how large the insertion or deletion is and where the change is made. The three potential effects of substitution mutations are shown in FIG. 5.18, while FIG. 5.19 shows the potential impact of insertion and deletion mutations. Mutation effects differ. Just as making changes to a recipe can lead to good, bad, or neutral effects in the resulting food, changes in the genome lead to diverse outcomes. Some mutations are helpful to a cell and increase its likelihood of survival—for instance, the mutation might help the cell better manage a certain environmental stress. Other mutations can be detrimental to a cell and compromise survival. Still others are neutral, having no impact on the cell’s phenotype. Silent Mutations Mutations that do not change the amino acid sequence of a protein are called silent mutations. Recall that the genetic code relies on codons to direct the production of a protein during translation. The genetic code is redundant–– meaning several different codons encode the same amino acid. Redundant codons tend to differ at the third, or so-called “wobble,” position of their FIGURE 5.18 Three potential effects of base- Normal Mutation TTT TTT TTT TTT Base changes in DNA template None TTC TGT ATT Resulting codon in mRNA AAA AAG ACA UAA Translated as Lysine Lysine Threonine Stop Wild-type (normal) Silent Missense Nonsense Original DNA Effect substitution mutations A base-substitution mutation results in a single nucleotide change in the DNA. The DNA is eventually transcribed into mRNA and the mRNA is translated into a protein. In a silent mutation, the resulting amino acid is not changed. In a missense mutation the new codon encodes the wrong amino acid. A nonsense mutation occurs if the change leads to a stop signal instead of an amino acid. Mutations M05_NORM8290_01_SE_C05.indd 145 145 24/08/17 10:16 AM Original DNA Normal Single insertion Single deletion TACTTTGAATATACT TACTTTGAATATACT TACTTTGAATATACT None Insert Adenine (A) Remove Thymine (T) TACTTTGAATATACT TACATTTGAATATACT TACTTTGAATATACT AUG AAA CUU AUA UGA AUG UAA ACU UAU AUG A Mutation Resulting DNA Resulting mRNA AUG AAC UUA UAU GA Read as Methionine Lysine Leucine Isoleucine Stop Effect Wild-type (normal) Methionine Stop Premature stop Methionine Asparagine Leucine Tyrosine Completely altered protein FIGURE 5.19 Insertion and deletion mutations Insertions and deletions can shift the mRNA reading frame and lead to drastic changes in the resulting protein. Critical Thinking Why are base-substitution mutations usually less troublesome to a cell than an insertion or deletion? triplet sequence. Therefore, a nucleotide change at the wobble position tends to be silent because it is unlikely to change which amino acid is added to the protein being made. For example, the amino acid arginine would be added to the growing protein if the ribosome encountered the codons CGU, CGC, CGA, or CGG (see Table 5.4 on page 14). In this case, a mutation in the third position of the codon would have no effect on the amino acid encoded and therefore would not alter the protein being assembled. This built-in redundancy limits the impact of base-substitution mutations and is an important protective feature of the genetic code. Occasionally a base-substitution mutation can be corrected by another base substitution; in effect, the error is corrected by another error. This is called a reversion mutation, or back-mutation, since the second mutation caused the DNA to revert back to the original sequence. This may be one of the few situations in which two wrongs really do make a right! Nonsense, Missense, and Frameshift Mutations A mutation that modifies the meaning of a codon can be a dangerous scenario for a cell. For example, if a base-substitution mutation changed the codon CGA (which encodes arginine) to UGA (a stop signal), the ribosome would prematurely stop building the protein when it came across the mutated codon. As a result, the cell would end up with an incomplete protein that likely can’t perform its job. A mutation that causes a codon to go from encoding an amino acid to encoding a stop signal is called a nonsense mutation. In studies using E. coli, it was estimated that about 3 percent of all substitution mutations are 146 CHAPTER 5 Genetics M05_NORM8290_01_SE_C05.indd 146 24/08/17 10:16 AM nonsense mutations.11 In comparison, a missense mutation, in which the meaning of the codon is changed in a way that the wrong amino acid is added to the growing protein, is far more common. For example, if the codon CGU (arginine) was changed to UGU (serine), the protein’s amino acid sequence would be altered. So far we have discussed the consequences of substituting one base for another, but bases can also be inserted or deleted. Inserting or deleting bases from the coding region of a genetic sequence results in a frameshift mutation. Such mutations can be devastating for a cell because they tend to lead to a useless protein. For example, if you were told to decode the message THEBOYRANFORTHETOY by breaking it into sequential three letter chunks, the message would be decoded as: THE BOY RAN FOR THE TOY. Now, let’s insert one letter into the sequence. Doing this will give us: THEBOYARANFORTHETOY, which would decode as: THE BOY ARA NFO RTH ETO—gibberish. Similarly, cells follow decoding rules; they start decoding mRNA at a start codon and read it in triplet code to build a protein. If the reading frame is shifted by insertions or deletions, then the mRNA will direct the building of a jumbled protein that is usually useless to the cell. The impact of insertions and deletions is minimized if these mutations occur in multiples of three. For example, if we were to add three letters to the sequence THEBOYARABCNFORTHETOY it would be decoded as: THE BOY ARA BCN FOR THE TOY. While this sentence is not as coherent as the original, it still conveys part of the original meaning. The triple inser tion changed some words, but the reading frame itself was not entirely shifted. Likewise, in a gene, the insertion or deletion of bases in multiples of three (i.e., three, six, nine, etc.) can alter the final protein by adding or eliminating amino acids. Again, the consequences of such mutations always depend on the location and extent of the mutation. Mutations can be spontaneous or induced. Naturally occurring mutations are often referred to as spontaneous mutations. Most spontaneous mutations are either neutral or harmful to a cell. In the rare instance that a mutation is beneficial, the mutant strain (or the cells carrying the mutation) may have a survival advantage over nonmutated, or wild-type, strains in a particular environment. This survival advantage increases the chances for the mutant strain to pass the mutation to other generations. Over time, the mutation may become a standard trait in cell lines that are able to thrive in a particular environment. Spontaneous mutations are an important mechanism for evolution because they introduce genetic variation even in organisms that replicate asexually, like viruses and bacteria. It is estimated that in bacteria there is a spontaneous mutation in one out of every 10 billion base pairs. This sounds like a rare occurrence, but when you consider that under ideal conditions E. coli can divide about every 20 to 30 minutes, it means that one cell can generate a population of over 1 million cells in about 10 hours. Since E. coli has about 4 million base pairs in its genome, this means that in a population of about a million cells, at least 400 mutations will have occurred. This represents a tremendous opportunity for evolution in just 10 hours. Over time, enough mutations occur that eventually a cell will make an unexpectedly lucky mistake that confers a fabulous advantage. 11 Lee, H., Popodi, E., Tang, H., and Foster, P. (2012) Rate and molecular spectrum of spontaneous mutations in the bacterium Escherichia coli as determined by whole-genome sequencing. PNAS, vol. 109, no. 41, pp. E2774–E2783. TRAINING TOMORROW’S HEALTH TEAM The New Field of Genetics Nursing Advances in genetics and the beginning of personalized medicine led to the development of a new specialization in health care called genetics nursing. A genetics nurse is a licensed professional with additional training in genetics. These nurses work closely with physicians and genetics counselors in practices that diagnose or manage genetically based disorders. Genetics nurses help assess the contributions of genetic factors to disease, collect and analyze samples for genetic testing, and educate patients and their families about how genetic factors contribute to disease. Some genetics nurses specialize in prenatal care, assisting with genetic screenings and sharing results of such tests with patients. They also help patients understand the risk of passing a specific genetic disorder to a child, and how to care for a child with a genetic disorder. Most genetics nurses have a bachelor’s of nursing degree and after being licensed to practice nursing they gain their genetics certification through the Genetic Nursing Credentialing Commission (GNCC). Mutations M05_NORM8290_01_SE_C05.indd 147 147 24/08/17 10:16 AM Control Liver extract Experimental tube Liver extract Suspected mutagen Culture of his– Salmonella Medium lacking histidine After incubation Effect A few colonies may appear due to spontaneous reversion to his+ Number of colonies is higher than expected for spontaneous mutation to his+ FIGURE 5.20 Ames test In the Ames test a sample of his − Salmonella is exposed to a test chemical and a control sample is not exposed to the test chemical. The addition of liver enzymes provides a more accurate simulation of how the chemical being tested may “appear” once it is chemically processed by the body. Both samples are plated onto a medium that is lacking the amino acid histidine. If the tested chemical causes the bacteria to mutate at a rate higher than spontaneous mutation, the test plate will have significantly more colonies on it than the control plate. In such a case, the test chemical is a potential mutagen and possibly a carcinogen. Critical Thinking Would you expect this experiment to work if the bacteria were grown on media that contained the amino acid histidine? Explain your reasoning. Mutagens and Carcinogens Mutagens are chemical, physical, or biological agents that increase the rate of mutation. Chemical mutagens include organic or inorganic agents. These agents cause mutations by a variety of methods, such as inducing breaks in DNA, modifying bases, or promoting frameshift mutations by directly inserting themselves into the DNA. Examples of chemical mutagens include arsenic, asbestos, alcohol, and a variety of compounds in tobacco smoke. Physical mutagens induce DNA damage similarly to how chemical mutagens do, but they include radiation such as ultraviolet (UV) light, X-rays, and radioactive gamma rays. Biological mutagens are agents that can introduce genetic change through recombination, which is an exchange of genetic material that leads to new genetic combinations. Transposons and certain viruses can act as biological mutagens (these agents are discussed further in the last section of the chapter). Many mutagens cause a rate of mutation that promotes the development of cancers; such mutagens are called carcinogens. Ames Test Natural and synthetic compounds have the potential to be mutagens, and many mutagens are carcinogens. Being able to test if a substance has harmful effects on humans is increasingly important, given that the CDC estimates that about 49 percent of Americans take at least one prescription drug in any given month, and there are over 85,000 industrial chemicals currently in use that people may come into contact with, including flame retardants in upholstery or clothing, plastic drink and food containers, pollution in the air we breathe, etc. While animal studies are often used for safety testing a variety of agents, these studies are expensive and inefficient. The Ames test was developed to quickly identify mutagens that alter DNA by either base substitutions or by frameshift mutations. It relies on strains of Salmonella typhimurium bacteria that cannot make the amino acid histidine (called his - strains). This amino acid is essential for growth; cells that cannot make their own histidine must get it from their environment. Like all cells, his - bacteria experience spontaneous mutations, and as a result they may regain their ability to make histidine (revert to his + ). Normal cells have repair mechanisms (discussed next) that would address these mutations. However, the mutations in the his - Ames strains are not repaired, because these strains lack DNA repair tools. This deficiency allows for the detection of reversion mutations, since only his + reversion mutants will grow on the histidine-free agar used in the test (FIG. 5.20). If his - strains are grown in the presence of a mutagen, they experience more mutations and consequently the rate of his + reversion is greater than that produced by spontaneous mutation. Therefore, when his - bacteria are exposed to a chemical and experience a reversion rate above that seen for spontaneous mutation, the tested chemical is regarded as a mutagen. DNA proofreading and repair mechanisms protect the stability of the genome. Although DNA polymerases proofread the DNA they build and fix detected errors, they may still make mistakes that don’t get corrected. Errors made by DNA polymerases during replication are an important contributor to the total spontaneous mutation rate, which is estimated to be one error in every 10 billion base pairs. However, errors are also introduced outside of replication, whenever DNA is damaged by a variety of agents such as mutagens or reactive intermediates from cellular metabolism. If cells did not have the ability to find and repair errors, then the rate of spontaneous mutations could be as much as one thousand times higher.12 During excision repair, specialized enzymes clip out damaged or mismatched nucleotides, and then DNA polymerase I lays down new nucleotides to repair the DNA. The enzyme ligase, which was used to glue Okazaki fragments 12 Kunkel, T. A. and Erie, D. A. (2005) DNA mismatch repair. Annual Review of Biochemistry, vol. 74, pp. 681–710. 148 CHAPTER 5 Genetics M05_NORM8290_01_SE_C05.indd 148 24/08/17 10:16 AM FIGURE 5.21 Excision repair UV radiation can lead to the formation of thymine dimers, which form a bulge in the DNA. Excision repair factors are recruited to the bulge to clip out the damaged section of the DNA strand, leaving the opposite strand untouched. DNA polymerase I and ligase then repair the gap. Thymine dimer dimer Thymine 1 UV radiation causes neighboring thymines to cross-link, causing a bulge in the DNA. T TT A A DNA Excision complex 2 Critical Thinking People with xeroderma pigmentosum have faulty DNA repair mechanisms and can’t go in the sun because their skin cells can’t repair DNA damage inflicted by UV radiation. However, these patients have a short life span even if they stay out of the sun. How might this be explained? Excision complex removes bulging section of DNA. Nucleotides are excised from only one strand. DNA polymerase I 3 DNA polymerase I adds new deoxyribonucleotides to fill the gap. 4 Ligase repairs nicks in the sugar–phosphate backbone. DNA ligase together in DNA replication, also glues the nicks in the DNA sugar–phosphate backbone, so that the repair patch is seamless (FIG. 5.21). Excision repair is especially active in fixing UV radiation damage in DNA. UV light has the ability to cause neighboring thymine bases in DNA to cross-link and form a bulge in the DNA. These thymine dimers interfere with DNA replication and transcription unless they are removed and repaired. The human genetic disorder xeroderma pigmentosum is a testimony to the importance of DNA repair mechanisms. When patients with this disorder are exposed to sunlight, a common and powerful source of UV radiation, their skin cells experience DNA damage that cannot be repaired, ultimately leading to skin cancer and death at an early age. Whenever the rate of DNA damage outpaces the rate of DNA repair, mutations accumulate in a cell and have detrimental effects. In single-celled organisms, this usually results in death. In animals, accumulated mutations often manifest as cancers. While DNA repair in higher eukaryotes is more involved than in prokaryotes, these repair mechanisms are well conserved across prokaryotes and eukaryotes. BUILD YOUR FOUNDATION 34. Why would a substitution mutation likely have less impact on a cell than a frameshift or deletion mutation? 35. What are spontaneous mutations, how do they come about, and how do they contribute to evolution? 36. Name three classes of mutagens and discuss how they induce mutations. 37. How is a his − strain of S. typhimurium used in the Ames test to help detect mutagens? 38. What are thymine dimers, and how do cells deal with them? What happens if thymine dimers are not removed? QUICK QUIZ Build your foundation by answering the Quick Quiz: scan this code or visit the Mastering Microbiology Study Area to quiz yourself. Mutations M05_NORM8290_01_SE_C05.indd 149 149 24/08/17 10:16 AM GENETIC VARIATION WITHOUT SEXUAL REPRODUCTION After reading this section, you should be able to: 35 Explain what plasmids are and describe why they are important in nature and in the laboratory. 36 Compare horizontal and vertical gene transfer. 37 Summarize what conjugation is and how it contributes to genetic diversity. 38 Define recombination. 39 Describe transformation and how Griffith’s experiments demonstrated this process. 40 Compare and contrast specialized and generalized transduction, and describe how they lead to genetic variation in bacteria. 41 Describe the two main categories of transposons, and how they can impact a cell’s genetic landscape. The Case of the Spreading Superbug CLINICAL CASE NCLEX HESI TEAS Practice applying what you know clinically: scan this code or visit the Mastering Microbiology Study Area to watch Part 3 and practice for future exams. Horizontal gene transfer allows bacteria to share genes without a cell division event. Vertical gene transfer occurs when cells pass their genetic information to the next generation (from parent cell to offspring) as a result of sexual or asexual cell division. In contrast, horizontal gene transfer passes genetic information between cells by a process independent of cell division, and therefore separate from reproduction. It enables cells that are related or not related to share genetic information. Horizontal gene transfer may even occur across different phylogenetic domains. For example, there is evidence that the bacterium Neisseria gonorrhoeae, which causes gonorrhea in humans, has acquired some human gene sequences.13 The integration of bacterial DNA into human cells has also been documented, and may promote the development of certain cancers.14 Some key mechanisms of horizontal gene transfer are conjugation, transformation, transduction, and the actions of transposons. Plasmids Plasmids are nonchromosomal DNA segments found in bacteria and a number of eukaryotic cells; they are commonly shared among cells by horizontal gene transfer mechanisms. Because they are easily manipulated in the laboratory, molecular biologists often use plasmids to generate cell lines that make useful products for medical applications. (See Chapter 14 and the Bench to Bedside in this chapter for more on plasmids in biomedical applications.) These small usually circular segments of double-stranded DNA tend to carry only a few genes. Plasmid genes are rarely essential to the cell; instead, they tend to confer advantages such as the ability to make toxins, protection against antibiotics, or the ability to perform conjugation. Conjugation Conjugation is the closest thing to sex that bacteria have, but it is not sexual reproduction. In conjugation, a bacterium that carries a fertility plasmid forms a small hollow tube called a pilus, which attaches to a neighboring bacterial cell that lacks a fertility plasmid. The pilus serves as a bridge for transferring a copy of the fertility plasmid to the cell lacking it (FIG. 5.22 left). Then the pilus is dismantled and the cells separate. Assuming a complete copy of the fertility plasmid transfers, the recipient cell can then initiate conjugation with cells that lack fertility plasmids. From a medical standpoint, the troubling part of conjugation is that fertility plasmids often carry genes that endow cells with drug resistance or the ability to produce toxins. Of further concern is that the partners in conjugation do not have to be the same bacterial species, which facilitates the widespread sharing of antibiotic-resistance genes and other potentially dangerous genetic tools across bacterial species. Occasionally the fertility plasmid will merge with the bacterial cell’s chromosome and cease to be an independent plasmid in the cytoplasm. When this occurs, the bacterial cell is called a high-frequency recombination (Hfr) strain (FIG. 5.22 right). This process, called recombination, is important in nature and is also used as a molecular biology tool that allows researchers to alter the genetic landscape of a cell. Hfr cells share genetic material with cells that lack a fertility plasmid. The amazing thing about Hfr strains is that they can pass on copied segments of DNA 13 Anderson, M. and Steven, H. S. (2011) Opportunity and means: Horizontal gene transfer from the human host to a bacterial pathogen. mBio, vol. 2, issue 1, pp. e00005–e00011. 14 Riley, D. R., Sieber, K. B., Robinson, K. M., White, J. R., Ganesan, A., Nourbakhsh, S., and Dunning Hotopp, J. C. (2013) Bacteria-human somatic cell lateral gene transfer is enriched in cancer samples. PLOS Computational Biology, vol. 9, issue 6. 150 CHAPTER 5 Genetics M05_NORM8290_01_SE_C05.indd 150 24/08/17 10:16 AM F factor Hfr strain Cell with F factor Cell without F factor left bacterium 1 The carries a fertility Hfr cell Cell without F plasmid left bacterium has F 1 The plasmid integrated into plasmid (F factor). its genome, making it an Hfr strain. F factor F factor integrated into chromosome Bacterial chromosome Pilus with fertility 2 Bacterium plasmid builds a pilus Hfr bacterium builds a 2 The pilus to attach to the dissolves and 3 Pilus both cells now DNA integrates 3 Donated into new host’s chromosome, to attach to the neighboring cell; F plasmid is transferred across the pilus. contain F plasmid. neighboring F cell. The F plasmid along with part of the host chromosomal DNA is transferred across the pilus into the neighboring cell and the pilus dissolves. The amount of DNA transferred depends on how long the pilus is maintained. converting it into an Hfr strain like the donor. FIGURE 5.22 Conjugation beyond the usual set of genes found on the fertility plasmid. In fact, if the pilus is maintained for a sufficient amount of time (about 100 minutes in E. coli), the Hfr strain can pass a complete copy of its genome to the recipient cell. This has tremendous implications for how bacteria evolve. Transformation Another horizontal gene transfer process is called transformation. Here bacteria are genetically altered when they take up DN