Module 3: The Cell (Week 3) PDF
Document Details
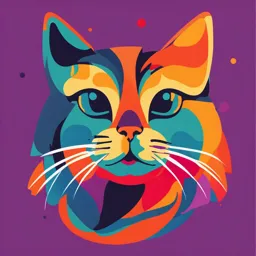
Uploaded by LuxuriantLutetium
Mindanao State University
Tags
Summary
This document is a module on the cell, covering its structure, functions, and related concepts for cell biology. The text includes a section on cell respiration and cell division.
Full Transcript
B101 General Zoology Module 3: The Cell (Week 3) Introduction Cells are the basic structural and functional units of all living organisms. Eukaryotic cells differ from the prokaryotic cells of bacteria and archaebacteria...
B101 General Zoology Module 3: The Cell (Week 3) Introduction Cells are the basic structural and functional units of all living organisms. Eukaryotic cells differ from the prokaryotic cells of bacteria and archaebacteria in several respects, the most distinctive of which is the presence of a membrane- bound nucleus containing chromosomes that carry the hereditary material. Cells are surrounded by a plasma membrane that regulates the flow of molecular traffic between the cell and its surroundings. The nucleus, enclosed by a double membrane, contains chromatin and one or more nucleoli. Outside the nuclear envelope is cell cytoplasm, subdivided by a membranous network, the endoplasmic reticulum. Among the organelles within cells are the Golgi complex, mitochondria, lysosomes, and other membrane-bound vesicles. The cytoskeleton is composed of microfilaments (actin), microtubules (tubulin), and intermediate filaments (several types). Cilia and flagella are hairlike, motile appendages that contain microtubules. Ameboid movement by pseudopodia operates by means of actin microfilaments. Tight junctions, desmosomes, and gap junctions are structurally and functionally distinct connections between cells. Membranes in the cell are composed of a phospholipid bilayer and other materials including cholesterol and proteins. Hydrophilic ends of the phospholipid molecules are on the outer and inner surfaces of membranes, and the fatty acid portions are directed inward, toward each other, to form a hydrophobic core. Substances can enter cells by diffusion, mediated transport, and endocytosis. Osmosis is diffusion of water through a selectively permeable membrane as a result of osmotic pressure. Solutes to which the membrane is impermeable require a transporter or permease molecule to traverse the membrane. Permease-mediated systems include facilitated diffusion (in the direction of a concentration gradient) and active transport (against a concentration gradient, which requires energy). Endocytosis includes bringing droplets (pinocytosis, potocytosis) or particles (phagocytosis) into the cell. In exocytosis the process of endocytosis is reversed. Cell division in eukaryotes includes mitosis, the division of the nuclear chromosomes, and cytokinesis, the division of the cytoplasm. Mitosis itself is only a small part of the total cell cycle. In interphase, G1, S, and G2 periods are recognized, and the S period is the time when DNA is synthesized (the chromosomes are replicated). Replicated chromosomes are each held together by a centromere. In prophase, replicated chromosomes condense into recognizable bodies. A spindle forms between the centrosomes as they separate to opposite poles of the cell. At the end of prophase the nuclear envelope disintegrates, and the kinetochores of each chromosome become attached to both centrosomes by microtubules (kinetochore fibers). At metaphase the sister chromatids are moved to the center of the cell. At anaphase the centromeres divide, and one of each kind of chromosome is pulled toward the centrosome by the attached kinetochore fiber. At telophase the chromosomes gather in the position of the nucleus in each cell and revert to a diffuse chromatin network. A nuclear membrane reappears, and cytokinesis occurs. Cells divide rapidly during embryonic development, then more slowly with age. Some cells continue to divide throughout the life of an animal to replace cells lost by attrition and wear, whereas others, such as nerve and muscle cells, complete their division during early development and never divide again. Some cells undergo a programmed cell death, or apoptosis. Topic 3. Cells as Units of Life Activating Prior Knowledge Reflect on the learning outcomes above and complete the table below. Write only what you know about the given topics. Be honest with your answers. Leaning Outcomes Topic What you know Illustrate the structural The cell parts of the typical animal 1. Cell structure cell Separate and categorize Cell functions cell parts according to the common functions that they perform Describe organisms and Classification of familiarize the taxonomic Nomenclature of Animals categories based on certain characteristics Explain the basic 2. Cell respiration mechanisms of cell 2.1. Anaerobic respiration respiration and cell division (Glycolysis) 2.2. aerobic respiration (Krebs Cycle and Electron transport system) Explain an discuss the basic 3. Cell reproduction process of cell 3.1. Mitosis reproduction and the 3.2. Meiosis central dogma of life 4. Central dogma of life (replication, transcription 4.1. Replication and translation) 4.2. Transcription 4.3. Translation Let’s learn some facts Prokaryotes and the Age of Cyanobacteria (Blue-Green Algae) The earliest bacterium-like organisms proliferated, giving rise to a great variety of forms, some of which were capable of photosynthesis. From these arose the oxygen-producing cyanobacteria approximately 3 billion years ago. Bacteria are called prokaryotes, meaning literally “before the nucleus.” They contain a single, large molecule of DNA not located in a membrane-bound nucleus, but found in a nuclear region, or nucleoid. The DNA is not complexed with histone proteins, and prokaryotes lack membranous organelles such as mitochondria, plastids, Golgi apparatus, and endoplasmic reticulum (Chapter 3). During cell division, the nucleoid divides and replicates of the cell’s DNA are distributed to the daughter cells. Prokaryotes lack the chromosomal organization and chromosomal (mitotic) division seen in animals, fungi, and plants. Bacteria and especially cyanobacteria ruled the earth’s oceans unchallenged for 1 to 2 billion years. The cyanobacteria reached the zenith of their success approximately 1 billion years BP, when filamentous forms produced great floating mats on the oceans’ surface. This long period of cyanobacterial dominance, encompassing approximately two-thirds of the history of life, has been called with justification the “age of blue-green algae.” Bacteria and cyanobacteria are so completely different from forms of life that evolved later that they were placed in a separate kingdom, Monera. Appearance of the Eukaryotes The eukaryotes (“true nucleus”; Figure 2-15) have cells with membrane-bound nuclei containing chromosomes composed of chromatin. Constituents of eukaryotic chromatin include proteins called histones and RNA, in addition to the DNA. Some nonhistone proteins are found associated with both prokaryotic DNA and eukaryotic chromosomes. Eukaryotes are generally larger than prokaryotes and contain much more DNA. Cellular division usually is by some form of mitosis. Within their cells are numerous membranous organelles, including mitochondria, in which the enzymes for oxidative metabolism are packaged. Eukaryotes include animals, fungi, plants, and numerous single-elled forms formerly known as “protozoans” or “protists.” Fossil evidence suggests that single-celled eukaryotes arose at least 1.5 million years ago (Figure 2-16). Because the organizational complexity of the eukaryotes is much greater than that of the prokaryotes, it is difficult to visualize how a eukaryote could have arisen from any known prokaryote. The American biologist Lynn Margulis and others have proposed that eukaryotes did not in fact arise from any single prokaryote but were derived from a symbiosis (“life together”) of two or more types of bacteria. Mitochondria and plastids, for example, each contain their own complement of DNA (apart from the nucleus of the cell), which has some prokaryotic characteristics. Nuclei, plastids, and mitochondria each contain genes encoding ribosomal RNA. Comparisons of the sequence of bases of these genes show that the nuclear, plastid, and mitochondrial DNAs represent distinct evolutionary lineages. Plastid and mitochondrial DNAs are closer in their evolutionary history to bacterial DNAs than to the eukaryotic nuclear DNA. Plastids are closest evolutionarily to cyanobacteria, and mitochondria are closest to another group of bacteria (purple bacteria), consistent with the symbiotic hypothesis of eukaryotic origins. Mitochondria contain the enzymes of oxidative metabolism, and plastids (a plastid with chlorophyll is a chloroplast) conduct photosynthesis. It is easy to see how a host cell that was able to accommodate such guests in its cytoplasm would have had enormous evolutionary success. Eukaryotes may have originated more than once. The first eukaryotes were undoubtedly unicellular, and many were photosynthetic autotrophs. Some of these forms lost their photosynthetic ability and became heterotrophs, feeding on the autotrophs and the prokaryotes. As the cyanobacteria were cropped, their dense filamentous mats began to thin, providing space for other organisms. Carnivores appeared and fed on herbivores. Soon a balanced ecosystem of carnivores, herbivores, and primary producers appeared. By freeing space, cropping herbivores encouraged a greater diversity of producers, which in turn promoted the evolution of new and more specialized croppers. An ecological pyramid developed with carnivores at the top of the food chain (see figure below). The burst of evolutionary activity that followed at the end of the Precambrian period and beginning of the Cambrian period was unprecedented. Some investigators hypothesize that the explanation for the “Cambrian explosion” lies in the accumulation of oxygen in the atmosphere to a critical threshold level. Larger, multicellular animals required the increased efficiency of oxidative metabolism; these pathways could not be supported under conditions of limiting oxygen concentration. Cells are the fabric of life. Even the most primitive cells are enormously complex structures that form the basic units of all living organisms. All tissues and organs are composed of cells. In a human an estimated 60 trillion cells interact, each performing its specialized role in an organized partnership. In single-celled organisms all the functions of life are performed within the confines of one microscopic package. There is no life without cells. The idea that the cell represents the basic structural and functional unit of life is an important unifying concept of biology. With the exception of some eggs, which are the largest cells (in volume) known, cells are small and mostly invisible to the unaided eye. Consequently, our understanding of cells paralleled technical advances in the resolving power of microscopes. The Dutch microscopist A. van Leeuwenhoek sent letters to the Royal Society of London containing detailed descriptions of the numerous organisms he had observed using high-quality single lenses that he had made (1673 to 1723). In the early nineteenth century, the improved design of microscopes permitted biologists to see separate objects only one um apart. This advance was quickly followed by new discoveries that laid the groundwork for the cell theory—a theory stating that all living organisms are composed of cells. In 1838 Matthias Schleiden, a German botanist, announced that all plant tissue was composed of cells. A year later one of his countrymen, Theodor Schwann, described animal cells as being similar to plant cells, an understanding that had been long delayed because animal cells are bounded only by a nearly invisible plasma membrane rather than a distinct cell wall characteristic of plant cells. Schleiden and Schwann are thus credited with the unifying cell theory that ushered in a new era of productive exploration in cell biology. In 1840 J. Purkinje introduced the term protoplasm to describe cell contents. Protoplasm was at first thought to be a granular, gel-like mixture with special and elusive life properties of its own; cells were viewed as bags of thick soup containing a nucleus. Later the interior of cells became increasingly visible as microscopes were improved and better tissue-sectioning and staining techniques were introduced. Rather than being a uniform granular soup, a cell’s interior is composed of numerous cellular organelles, each performing a specific function in the life of a cell. Today we realize that the components of a cell are so highly organized, structurally and functionally, that describing its contents as “protoplasm” is like describing the contents of an automobile engine as “autoplasm.” Prokaryotic and Eukaryotic Cells We already described the radically different cell plan of prokaryotes and eukaryotes. A fundamental distinction, expressed in their names, is that prokaryotes lack the membrane-bound nucleus present in all eukaryotic cells. Among other differences, eukaryotic cells have many membranous organelles (specialized structures that perform particular functions within cells) (Table 3-1). Despite these differences, which are of paramount importance in cell studies, prokaryotes and eukaryotes have much in common. Both have DNA, use the same genetic code, and synthesize proteins. Many specific molecules such as ATP perform similar roles in both. These fundamental similarities imply common ancestry. Components of Eukaryotic Cells and Their Functions Typically, eukaryotic cells are enclosed within a thin, selectively permeable cell membrane (Figure 3-4). The most prominent organelle is the spherical or ovoid nucleus, enclosed within two membranes to form the double-layered nuclear envelope (Figure 3-4). The region outside the nucleus is regarded as cytoplasm. Within the cytoplasm are many organelles, such as mitochondria, Golgi complexes, centrioles, and endoplasmic reticulum. Plant cells typically contain plastids, some of which are photosynthetic organelles, and plant cells bear a cell wall containing cellulose outside the cell membrane. The fluid-mosaic model is the currently accepted concept of cell membranes. By electron microscopy, the cell membrane appears as two dark lines, each approximately 3 nm thick, at each side of a light zone (Figure 3-5). The entire membrane is 8 to 10 nm thick. This image is the result of a phospholipid bilayer, two layers of phospholipid molecules, all oriented with their water- soluble ends toward the outside and their fat-soluble portions toward the inside of the membrane (Figure 3-6). An important characteristic of the phospholipid bilayer is that it is liquid, giving the membrane flexibility and allowing the phospholipid molecules to move sideways freely within their own monolayer. Molecules of cholesterol are interspersed in the lipid portion of the bilayer (Figure 3-6). They make the membrane even less permeable and decrease its flexibility. Glycoproteins (proteins with carbohydrates attached) are essential components of cell membranes. Some of these proteins catalyze the transport of substances such as negatively charged ions across the membrane. Others act as specific receptors for various molecules or as highly specific markings. For example, the self/nonself recognition that enables the immune system to react to invaders (Chapter 37) is based on proteins of this type. Some aggregations of protein molecules form pores through which small polar molecules may enter. Like the phospholipid molecules, most of the glycoproteins can move laterally in the membrane, although more slowly. Nuclear envelopes contain less cholesterol than cell membranes, and pores in the envelope (Figure 3-7) allow molecules to move between nucleus and cytoplasm. Nuclei contain chromatin, a complex of DNA, basic proteins called histones, and nonhistone protein. Chromatin carries the genetic information, the code that results in most of the components characteristic of the cell after transcription and translation. Nucleoli are specialized parts of certain chromosomes that stain in a characteristically dark manner. They carry multiple copies of the DNA information to synthesize ribosomal RNA. After transcription from DNA, ribosomal RNA combines with protein to form a ribosome, detaches from the nucleolus, and passes to the cytoplasm through pores in the nuclear envelope. The outer membrane of the nuclear envelope is continuous with extensive membranous elements in the cytoplasm called endoplasmic reticulum (ER) (Figures 3-7 and 3-8). The space between the membranes of the nuclear envelope communicates with channels (cisternae) in the ER. The ER is a complex of membranes that separates some of the products of the cell from the synthetic machinery that produces them, apparently functioning as routes for transport of proteins within the cell. Membranes of the ER may be covered on their outer surfaces with ribosomes and are thus designated rough ER, or they may lack ribosomal covering and be called smooth ER. Smooth ER functions in synthesis of lipids and phospholipids. Protein synthesized by ribosomes on rough ER enters the cisternae and from there is transported to the Golgi apparatus or complex. The Golgi complex (Figures 3-9 and 3-10) is composed of a stack of membranous vesicles that function in storage, modification, and packaging of protein products, especially secretory products. The vesicles do not synthesize protein but may add complex carbohydrates to the molecules. Small vesicles of ER containing protein pinch off and then fuse with sacs on the “forming face” of a Golgi complex. After modification, the proteins bud off vesicles on the “maturing face” of the complex (Figure 3-10). The contents of some of these vesicles may be expelled to the outside of the cell, as secretory products destined to be exported from a glandular cell. Others may contain digestive enzymes that remain in the same cell that produces them. Such vesicles are called lysosomes (literally “loosening body,” a body capable of causing lysis, or disintegration). Enzymes that they contain are involved in the breakdown of foreign material, including bacteria engulfed by the cell. Lysosomes also are capable of breaking down injured or diseased cells and worn-out cellular components. Their enzymes are so powerful that they kill the cell that formed them if the lysosome membrane ruptures. In normal cells the enzymes remain safely enclosed within the protective membrane. Lysosomal vesicles may pour their enzymes into a larger membrane-bound body containing an ingested food particle, the food vacuole or phagosome. Other vacuoles, such as contractile vacuoles of some single-celled organisms (p. 219), may contain only fluid and function to regulate ions and water. Mitochondria (sing., mitochondrion) (Figure 3-11) are conspicuous organelles present in nearly all eukaryotic cells. They are diverse in size, number, and shape; some are rod-like, and others are more or less spherical. They may be scattered uniformly through the cytoplasm, or they may be localized near cell surfaces and other regions where there is high metabolic activity. A mitochondrion is composed of a double membrane. The outer membrane is smooth, whereas the inner membrane is folded into numerous plate-like or finger-like projections called cristae (Figure 3-11), which increases internal surface area where chemical reactions take place. These characteristic features make mitochondria easy to identify among the organelles. Mitochondria are often called “powerhouses of the cell,” because enzymes located on the cristae carry out the energy-yielding steps of aerobic metabolism. ATP (adenosine triphosphate), the most important energy transfer molecule of all cells, is produced in this organelle. Mitochondria are self- replicating. They have a tiny, circular genome, much like the genomes of prokaryotes except that it is much smaller. It contains DNA that specifies some, but not all, of the proteins of the mitochondrion. Eukaryotic cells characteristically have a system of tubules and filaments that form the cytoskeleton (Figures 3-12 and 3- 13). These provide support and maintain the form of cells, and in many cells, they provide a means of locomotion and translocation of organelles within the cell. Microfilaments are thin, linear structures, first observed distinctly in muscle cells, where they are responsible for the ability of the cell to contract. They are made of a protein called actin. Several dozen other proteins are known that bind with actin and determine its configuration and behavior in particular cells. One of these is myosin, whose interaction with actin causes contraction in muscle and other cells. Actin microfilaments also provide a means for moving messenger RNA from the nucleus to particular positions within the cell. Microtubules, somewhat larger than microfilaments, are tubular structures composed of a protein called tubulin (Figure 3-13). They play a vital role in moving the chromosomes toward the daughter cells during cell division as will be seen later, and they are important in intracellular architecture, organization, and transport. In addition, microtubules form essential parts of the structures of cilia and flagella. Microtubules radiate out from a microtubule organizing center, the centrosome, near the nucleus. Centrosomes are not membrane bound. Within centrosomes are found a pair of centrioles (Figures 3-4 and 3-14), which are themselves composed of microtubules. Microtubules radiating from the centrioles form the aster. Each centriole of a pair lies at right angles to the other and is a short cylinder of nine triplets of microtubules. They replicate before cell division. Although cells of higher plants do not have centrioles, a microtubule organizing center is present. Intermediate filaments are larger than microfilaments but smaller than microtubules. There are five biochemically distinct types of intermediate filaments, and their composition and arrangement depend on the cell type in which they are found. Surfaces of Cells and Their Specializations The free surface of epithelial cells (cells that cover the surface of a structure or line a tube or cavity) sometimes bears either cilia or flagella (sing., cilium, flagellum). These are motile extensions of the cell surface that sweep materials past the cell. In many single-celled organisms and some small multicellular forms, they propel the entire organism through a liquid medium. Flagella provide the means of locomotion for male reproductive cells of most animals and many plants. Cilia and flagella have different beating patterns (see p. 653), but their internal structure is the same. With few exceptions, the internal structures of locomotory cilia and flagella are composed of a long cylinder of nine pairs of microtubules enclosing a central pair (see Figure 11- 3). At the base of each cilium or flagellum is a basal body (kinetosome), which is identical in structure to a centriole. Many cells move neither by cilia nor flagella but by ameboid movement using pseudopodia. Some groups of protozoa (p. 217), migrating cells in embryos of multicellular animals, and some cells of adult multicellular animals, such as white blood cells, show ameboid movement. Cytoplasmic streaming through the action of actin microfilaments extends a lobe (pseudopodium) outward from the surface of the cell. Continued streaming in the direction of the pseudopodium brings cytoplasmic organelles into the lobe and accomplishes movement of the entire cell. Some specialized pseudopodia have cores of microtubules (p. 218), and movement is effected by assembly and disassembly of the tubular rods. Cells covering the surface of a structure (epithelial cells) or cells packed together in a tissue may have specialized junctional complexes between them. Nearest the free surface, the membranes of two cells next to each other appear to fuse, forming a tight junction (Figure 3-15). Tight junctions function as seals to prevent the passage of molecules between cells from one side of a layer of cells to another, because there is usually a space of about 20 nm between the cell membranes of adjacent cells. Tight junctions between intestinal cells, for example, force molecules absorbed from the intestinal contents to pass through the epithelial cells, rather than between them. At various points beneath tight junctions, small ellipsoid discs occur, just within the cell membrane in each cell. These appear to act as “spot-welds” and are called desmosomes. From each desmosome a tuft of intermediate filaments extends into the cytoplasm, and linker proteins extend through the cell membrane into the intercellular space to bind the discs together. Desmosomes are not seals but seem to increase the strength of the tissue. Gap junctions, rather than serving as points of attachment, provide a means of intercellular communication. They form tiny canals between cells, so that their cytoplasm becomes continuous, and small molecules can pass from one cell to the other. Gap junctions may occur between cells of epithelial, nervous, and muscle tissues. Another specialization of the cell surfaces is the lacing together of adjacent cell surfaces where the cell membranes of the cells in- fold and interdigitate very much like a zipper. They are especially common in the epithelial cells of kidney tubules. The distal or apical boundaries of some epithelial cells, as seen by electron microscopy, show regularly arranged microvilli. They are small, fingerlike projections consisting of tube-like evaginations of the cell membrane with a core of cytoplasm (Figure 3-16). They are seen clearly in the lining of the intestine where they greatly increase the absorptive and digestive surface. Such specializations appear as brush borders by light microscopy. Let’s have a break to review. Activity 1. Refer to the notes above. Write all the parts or organelles found exclusively in prokaryotic organisms and parts or organelles found exclusively in eukaryotic cells or organisms. Write your answers in the table provided. Write a short description of the function of each cell part or organelle. This will be helpful in the assessment activity later on. PROKARYOTIC CELL EUKARYOTIC CELLL Eg. Nucleus – control center of cell Nucleus – control center of the cell Membrane Function The incredibly thin, yet sturdy, plasma membrane that encloses every cell is vitally important in maintaining cellular integrity. Once believed to be a rather static entity that defined cell boundaries and kept cell contents from spilling out, the plasma membrane (also called the plasmalemma) is a dynamic structure having remarkable activity and selectivity. It is a permeability barrier that separates the interior from the external environment of the cell, regulates the vital flow of molecular traffic into and out of the cell, and provides many of the unique functional properties of specialized cells. Membranes inside the cell surround a variety of organelles. Indeed, the cell is a system of membranes that divide it into numerous compartments. Someone has estimated that if all membranes present in one gram of liver tissue were spread out flat, they would cover 30 square meters! Internal membranes share many of the structural features of plasma membranes and are the site for many, perhaps most, of the cell’s enzymatic reactions. A plasma membrane acts as a selective gatekeeper for the entrance and exit of the many substances involved in cell metabolism. Some substances can pass through with ease, others enter slowly and with difficulty, and still others cannot enter at all. Because conditions outside the cell are different from and more variable than conditions within the cell, it is necessary that the passage of substances across the membrane be rigorously controlled. We recognize three principal ways that a substance may traverse the cell membrane: (1) by diffusion along a concentration gradient; (2) by a mediated transport system, in which the substance binds to a specific site that in some way assists it across the membrane; and (3) by endocytosis, in which the substance is enclosed within a vesicle that forms on and detaches from the membrane surface to enter the cell. Diffusion and Osmosis Diffusion is a movement of particles from an area of high concentration to an area of lower concentration of the particles or molecules, thus tending to equalize the concentration throughout the area of diffusion. If a living cell surrounded by a membrane is immersed in a solution having a higher concentration of solute molecules than the fluid inside the cell, a concentration gradient instantly exists between the two fluids. Assuming that the membrane is permeable to the solute, there is a net movement of solute toward the inside, the side having the lower concentration. The solute diffuses “downhill” across the membrane until its concentrations on each side are equal. Most cell membranes are selectively permeable, that is, permeable to water but variably permeable or impermeable to solutes. In free diffusion it is this selectiveness that regulates molecular traffic. As a rule, gases (such as oxygen and carbon dioxide), urea, and lipid-soluble solutes (such as hydrocarbons and alcohol) are the only solutes that can diffuse through biological membranes with any degree of freedom. Because many water-soluble molecules readily pass through membranes, such movements cannot be explained by simple diffusion. Sugars, as well as many electrolytes and macromolecules, are moved across membranes by carrier-mediated processes, which are described in the next section. If we place a membrane between two unequal concentrations of solutes to which the membrane is impermeable, water flows through the membrane from the more dilute to the more concentrated solution. The water molecules move across the membrane down a concentration gradient from an area where the water molecules are more concentrated to an area on the other side of the membrane where they are less concentrated. This is osmosis. We can demonstrate osmosis by a simple experiment in which we tie a selectively permeable membrane such as cellophane tightly over the end of a funnel. We fill the funnel with a salt solution and place it in a beaker of pure water so that the water levels inside and outside the funnel are equal. In a short time the water level in the glass tube of the funnel rises, indicating a net movement of water through the cellophane membrane into the salt solution (Figure 3-17). Inside the funnel are salt molecules, as well as water molecules. In the beaker outside the funnel are only water molecules. Thus, the concentration of water is less on the inside because some of the available space is occupied by the larger, non-diffusible salt molecules. A concentration gradient exists for water molecules in the system. Water diffuses from the region of greater concentration of water (pure water outside) to the region of lesser concentration (salt solution inside). As water enters the salt solution, the fluid level in the funnel rises. Gravity creates a hydrostatic pressure inside the osmometer. Eventually the pressure produced by the increasing weight of solution in the funnel pushes water molecules out as fast as they enter. The level in the funnel becomes stationary and the system is in equilibrium. The osmotic pressure of the solution is equivalent to the hydrostatic pressure necessary to prevent further net entry of water. The concept of osmotic pressure is not without problems. A solution reveals an osmotic “pressure” only when it is separated from solvent by a selectively permeable membrane. It can be disconcerting to think of an isolated bottle of salt solution as having “pressure” much as compressed gas in a bottle (hydrostatic pressure) would have. Furthermore, the osmotic pressure is really the hydrostatic pressure that must be applied to a solution to keep it from gaining water if the solution were separated from pure water by a selectively permeable membrane. Consequently, biologists frequently use the term osmotic potential rather than osmotic pressure. However, since the term “osmotic pressure” is so firmly fixed in our vocabulary, it is necessary to understand the usage despite its potential confusion. The concept of osmosis is very important in understanding how animals control their internal fluid and solute environment. For example, marine bony fishes maintain a solute concentration in their blood about one-third of that in seawater; they are hypoosmotic to seawater. If a fish swims into a river mouth and then up a freshwater stream, as salmon do, it would pass through a region where its blood solutes were equal in concentration to those in its environment (isosmotic), then enter fresh water, where its blood solutes were hyperosmotic to those in its environment. It must have physiological mechanisms to avoid net loss of water in the sea and gain of water in the river. Mediated Transport We have seen that the cell membrane is an effective barrier to the free diffusion of most molecules of biological significance. Yet it is essential that such materials enter and leave the cell. Nutrients such as sugars and materials for growth such as amino acids must enter the cell, and the wastes of metabolism must leave. Such molecules are moved across the membrane by special proteins called transporters or permeases. Permeases form a small passageway through the membrane, enabling the solute molecule to cross the phospholipid bilayer (Figure 3-18A). Permeases are usually quite specific, recognizing and transporting only a limited group of chemical substances or perhaps even a single substance. At high concentrations of solute, mediated transport systems show a saturation effect. This means simply that the rate of influx reaches a plateau beyond which increasing the solute concentration has no further effect on influx rate (Figure 3-18B). This is evidence that the number of transporters available in the membrane is limited. When all transporters become occupied by solutes, the rate of transport is at a maximum and it cannot be increased. Simple diffusion shows no such limitation; the greater the difference in solute concentrations on the two sides of the membrane, the faster the influx. Two distinctly different kinds of mediated transport mechanisms are recognized: (1) facilitated diffusion, in which the permease assists a molecule to diffuse through the membrane that it cannot otherwise penetrate, and (2) (2) active transport, in which energy is supplied to the transporter system to transport molecules in the direction opposite a concentration gradient (Figure 3-19). Facilitated diffusion therefore differs from active transport in that it sponsors movement in a downhill direction (in the direction of the concentration gradient) only and requires no metabolic energy to drive the transport system. In many animals facilitated diffusion aids in the transport of glucose (blood sugar) into body cells that oxidize it as a principal energy source for the synthesis of ATP. The concentration of glucose is greater in the blood than in the cells that consume it, favoring inward diffusion, but glucose is a water-soluble molecule that does not, by itself, penetrate the membrane rapidly enough to support the metabolism of many cells; the carrier system increases the inward flow of glucose. In active transport, molecules are moved uphill against the forces of passive diffusion. Active transport always involves the expenditure of energy (from ATP) because materials are pumped against a concentration gradient. Among the most important active transport systems in all animals are those that maintain sodium and potassium ion gradients between cells and the surrounding extracellular fluid or external environment. Most animal cells require a high internal concentration of potassium ions for protein synthesis at the ribosome and for certain enzymatic functions. The potassium ion concentration may be 20 to 50 times greater inside the cell than outside. Sodium ions, on the other hand, may be 10 times more concentrated outside the cell than inside. Both of these ionic gradients are maintained by the active transport of potassium ions into and sodium ions out of the cell. In many cells the outward pumping of sodium is linked to the inward pumping of potassium; the same transporter molecule does both. As much as 10% to 40% of all the energy produced by the cell is consumed by the sodium-potassium exchange pump (Figure 3-19). Endocytosis Endocytosis, the ingestion of material by cells, is a collective term that describes three similar processes, phagocytosis, potocytosis, and receptor-mediated endocytosis (Figure 3-20). They are pathways for specifically internalizing solid particles, small molecules and ions, and macromolecules, respectively. All require energy and thus may be considered forms of active transport. Phagocytosis, which literally means “cell eating,” is a common method of feeding among protozoa and lower metazoa. It is also the way in which white blood cells (leukocytes) engulf cellular debris and uninvited microbes in the blood. By phagocytosis, an area of the cell membrane, coated internally with actin-myosin, forms a pocket that engulfs the solid material. The membrane-enclosed vesicle then detaches from the cell surface and moves into the cytoplasm where its contents are digested by intracellular enzymes. Potocytosis is similar to phagocytosis except that small areas of the surface membrane are invaginated into cells to form tiny vesicles. The invaginated pits and vesicles are called caveolae (ka-vee’o-lee). Specific binding receptors for the molecule or ion to be internalized are concentrated on the cell surface of caveolae. Potocytosis apparently functions for intake of at least some vitamins, and similar mechanisms may be important in translocating substances from one side of a cell to the other (see “exocytosis,” following) and internalizing signal molecules, such as some hormones or growth factors. Receptor-mediated endocytosis is a specific mechanism for bringing large molecules within the cell. Proteins of the plasma membrane specifically bind particular molecules (referred to as ligands in this process), which may be present in the extracellular fluid in very low concentrations. The invaginations of the cell surface that bear the receptors are coated within the cell with a protein called clathrin; hence, they are described as clathrin-coated pits. As a clathrin-coated pit with its receptor-bound ligand invaginates and is brought within the cell, it is uncoated, the receptor and the ligand are dissociated, and the receptor and membrane material are recycled back to the surface membrane. Some important proteins and peptide hormones are brought into cells in this manner. Exocytosis Just as materials can be brought into the cell by invagination and formation of a vesicle, the membrane of a vesicle can fuse with the plasma membrane and extrude its contents to the surrounding medium. This is the process of exocytosis. This process occurs in various cells to remove undigestible residues of substances brought in by endocytosis, to secrete substances such as hormones (Figure 3-10), and to transport a substance completely across a cellular barrier, as we just mentioned. For example, a substance may be picked up on one side of the wall of a blood vessel by potocytosis, moved across the cell, and released by exocytosis. Assessment Activity This is a major activity that requires you to do the following quiz PLEASE REFER TO THE CLASSWORK IN GOOGLE CLASSROOM. MAKE SURE TO HAND IN OR SUBMIT AS DONE. Reminder. ! Before you proceed, make sure to complete the Assessment activity above in the Google classroom. Learning Activity. Read the reading material below. While reading, be guided by the following questions and answer the assessment activity in the Google classroom: 1. What is cellular respiration? 2. What is electron for? 3. How is energy generated? 4. What is the difference between aerobic an anaerobic respiration? Cellular Respiration How Electron Transport Is Used to Trap Chemical Bond Energy ATP is the one common energy denominator by which all cellular machines are powered, we are in a position to ask how this energy is captured from fuel substrates. This question directs us to an important generalization: all cells obtain their chemical energy requirements from oxidation-reduction reactions. This means that in the degradation of fuel molecules, hydrogen atoms (electrons and protons) are passed from electron donors to electron acceptors with a release of energy. A portion of this energy is trapped and used to form the high-energy bonds in ATP. Because they are so important, let us review what we mean by oxidation-reduction (“redox”) reactions. In these reactions there is a transfer of electrons from an electron donor (the reducing agent) to an electron acceptor (the oxidizing agent). As soon as the electron donor loses its electrons, it becomes oxidized. As soon as the electron acceptor accepts electrons, it becomes reduced (Figure 4-9). In other words, a reducing agent becomes oxidized when it reduces another compound, and an oxidizing agent becomes reduced when it oxidizes another compound. Thus, for every oxidation there must be a corresponding reduction. In an oxidation-reduction reaction the electron donor and electron acceptor form a redox pair: Electron donor e ̅ Ⴕ Electron acceptor Ⴕ Energy (reducing agent; (oxidizing agent; becomes oxidized) becomes reduced) When electrons are accepted by the oxidizing agent, energy is liberated because the electrons move to a more stable position. In a cell, electrons flow through a series of carriers. Each carrier is reduced by accepting electrons and then is reoxidized by passing electrons to the next carrier in the series. By transferring electrons stepwise in this manner, energy is gradually released, and a maximum yield of ATP is realized. Aerobic Versus Anaerobic Metabolism Ultimately, the electrons are transferred to a final electron acceptor. The nature of this final acceptor is the key that determines the overall efficiency of cellular metabolism. Heterotrophs (organisms that cannot synthesize their own food but must obtain nutrients from the environment, including animals, fungi, and many single-celled organisms) can be divided into two groups: aerobes, those that use molecular oxygen as the final electron acceptor, and anaerobes, those that employ some other molecule as the final electron acceptor. Life originated in the absence of oxygen, and the abundance of atmospheric oxygen was produced after photosynthetic organisms (autotrophs) evolved. Some strictly anaerobic organisms still exist and indeed play some important roles in specialized habitats. However, evolution has favored aerobic metabolism, not only because oxygen became available, but also because aerobic metabolism is vastly more efficient than anaerobic metabolism. In the absence of oxygen, only a very small fraction of the bond energy present in foodstuffs can be released. For example, when an anaerobic microorganism degrades glucose, the final electron acceptor (such as pyruvic acid) still contains most of the energy of the original glucose molecule. An aerobic organism on the other hand, using oxygen as the final electron acceptor, can completely oxidize glucose to carbon dioxide and water. Almost 20 times as much energy is released when glucose is completely oxidized as when it is degraded only to the stage of pyruvic acid. An obvious advantage of aerobic metabolism is that a much smaller quantity of foodstuffs is required to maintain a given rate of metabolism. Overview of Respiration Aerobic metabolism is more familiarly known as true cellular respiration, defined as the oxidation of fuel molecules with molecular oxygen as the final electron acceptor. The oxidation of fuel molecules describes the removal of electrons and not the direct combination of molecular oxygen with fuel molecules. Let us look at this process in general before considering it in more detail. Hans Krebs, the British biochemist who contributed so much to our understanding of respiration, described three stages in the complete oxidation of fuel molecules to carbon dioxide and water (Figure 4-10). In stage I, foodstuffs passing through the intestinal tract are digested into small molecules that can be absorbed into the circulation. There is no useful energy yield during digestion, which is discussed in Chapter 34. In stage II, most of the degraded foodstuffs are converted into two 3-carbon units (pyruvic acid) in the cell cytoplasm. The pyruvic acid molecules then enter mitochondria, where in another reaction they join with a coenzyme (coenzyme A) to form acetyl-CoA. Some ATP is generated in stage II, but the yield is small compared with that obtained in stage III of respiration. In stage III the final oxidation of fuel molecules occurs, with a large yield of ATP. This stage takes place entirely in mitochondria. Acetyl coenzyme A is channeled into the Krebs cycle where the acetyl group is completely oxidized to carbon dioxide. Electrons released from acetyl groups are transferred to special carriers that pass them to electron acceptor compounds in the electron transport chain. At the end of the chain the electrons (and the protons accompanying them) are accepted by molecular oxygen to form water. Glycolysis We begin our journey through the stages of respiration with glycolysis, a nearly universal pathway in living organisms that converts glucose into pyruvic acid. In a series of reactions, glucose and other 6-carbon monosaccharides are split into 3-carbon fragments, pyruvic acid (Figure 4-11). A single oxidation occurs during glycolysis, and each molecule of glucose yields two molecules of ATP. In this pathway the carbohydrate molecule is phosphorylated twice by ATP, first to glucose-6-phosphate (not shown in Figure 4-11) and then to fructose-1,6- diphosphate. The fuel has now been “primed” with phosphate groups in this uphill portion of glycolysis and is sufficiently reactive to enable subsequent reactions to proceed. This is a kind of deficit financing that is required for an ultimate energy return many times greater than the original energy investment. In the downhill portion of glycolysis, fructose-1,6-diphosphate is cleaved into two 3- carbon sugars, which undergo an oxidation (electrons are removed), with the electrons and one of the hydrogen ions being accepted by nicotinamide adenine dinucleotide (NAD, a derivative of the vitamin niacin) to produce a reduced form called NADH. NADH serves as a carrier molecule to convey high energy electrons to the final electron transport chain, where ATP will be produced. The two 3-carbon sugars next undergo a series of reactions, ending with the formation of two molecules of pyruvic acid (Figure 4-11). In two of these steps, a molecule of ATP is produced. In other words, each 3-carbon sugar yields two ATP molecules, and since there are two 3-carbon sugars, four ATP molecules are generated. Recalling that two ATP molecules were used to prime the glucose initially, the net yield up to this point is two ATP molecules. The 10 enzymatically catalyzed reactions in glycolysis can be summarized as: Glucose Ⴕ 2 ADP Ⴕ 2 Pi Ⴕ 2 NAD+ → 2 pyruvic acid Ⴕ 2 NADH Ⴕ 2 ATP Acetyl Coenzyme A: Strategic Intermediate in Respiration In aerobic metabolism the two molecules of pyruvic acid formed during glycolysis enter a mitochondrion. There, each molecule is oxidized, and one of the carbons is released as carbon dioxide (Figure 4-12). The 2-carbon residue condenses with coenzyme A to form acetyl coenzyme A (acetyl-CoA). Acetyl coenzyme A is a critically important compound. Its oxidation in the Krebs cycle (following) provides energized electrons to generate ATP, and it is a crucial intermediate in lipid metabolism. Krebs Cycle: Oxidation of Acetyl Coenzyme A Degradation (oxidation) of the 2-carbon acetyl group of acetyl coenzyme A occurs in a cyclic sequence called the Krebs cycle (also called citric acid cycle and tricarboxylic acid cycle [TCA cycle]) (Figure 4-13). Acetyl coenzyme A condenses with a 4-carbon acid (oxaloacetic acid), releasing coenzyme A to react again with pyruvic acid. Through a series of reactions the two carbons from the acetyl group are released as carbon dioxide, and oxaloacetic acid is regenerated. Hydrogen ions and electrons in the oxidations transfer to NAD and to FAD (flavine adenine dinucleotide, another electron acceptor), and a pyrophosphate bond is generated in the form of guanosine triphosphate (GTP). This high-energy phosphate readily transfers to ADP to form ATP. The overall products of the Krebs cycle are CO2, ATP, NADH, and FADH2: Acetyl unit Ⴕ 3 NAD+ Ⴕ FAD ႵADP Ⴕ Pi → 2 CO2 Ⴕ 3 NADH Ⴕ FADH2 ႵATP The molecules of NADH and FADH2 formed will yield 11 molecules of ATP when oxidized in the electron transport chain. The other molecules in the cycle behave as intermediate reactants and products which are continuously regenerated as the cycle turns. Electron Transport Chain Transfer of hydrogen ions and electrons from reduced NAD and FAD to the final electron acceptor, molecular oxygen, is accomplished in an elaborate electron transport chain embedded in the inner membrane of mitochondria (Figure 4-14). Each carrier molecule in the chain (labeled I to IV in Figure 4-14) is a large protein-based complex that accepts and releases electrons at lower energy levels than the carrier preceding it in the chain. As electrons pass from one carrier molecule to the next, free energy is released. Some of this energy drives the synthesis of ATP by setting up a H+ gradient across the mitochondrial membrane. At three points along the electron transport system, ATP production occurs by phosphorylation of ADP. By this means, oxidation of one NADH yields three ATP molecules. Reduced FAD from the Krebs cycle enters the electron transport chain at a lower level than NADH and so yields two ATP molecules. This method of energy capture is called oxidative phosphorylation because the formation of high-energy phosphate is coupled to oxygen consumption, and these reactions depend on the demand for ATP by other metabolic activities within the cell. How is ATP actually generated during oxidative phosphorylation? The most widely accepted explanation is a process called chemiosmotic coupling (Figure 4-14). According to this model, as electrons contributed by NADH and FADH2 are carried down the electron transport chain, they activate proton pumping channels which pump protons (hydrogen ions) outward and into the space between the two mitochondrial membranes. This causes the proton concentration outside to rise, producing a diffusion pressure that drives the protons back into the mitochondrion through special proton channels. These channels are ATP-forming protein complexes that use the inward passage of protons to induce the formation of ATP. Exactly how proton movement is coupled to ATP synthesis is not yet understood. Efficiency of Oxidative Phosphorylation We are now in a position to calculate the ATP yield from the complete oxidation of glucose (Figure 4-15). The overall reaction is: Glucose Ⴕ 2 ATP Ⴕ 36 ADP Ⴕ 36 P Ⴕ 6 O2 → 6 CO2 Ⴕ 2 ADP Ⴕ 36 ATP Ⴕ 6 H2O ATP has been generated at several points along the way (Table 4-1). The cytoplasmic NADH generated in glycolysis requires a molecule of ATP to fuel transport of each molecule of NADH into a mitochondrion; therefore, each NADH from glycolysis results in only two ATP (total of four), compared with the three ATP per NADH (total of six) formed within mitochondria. Accounting for the two ATP used in the priming reactions in glycolysis, the net yield may be as high as 36 molecules of ATP per molecule of glucose. (The yield of 36 ATP is a theoretical maximum because some of the H+ gradient produced by electron transport may be used for other functions, such as transporting substances in and out of the mitochondrion.) Overall efficiency of aerobic oxidation of glucose is about 38%, comparing very favorably with human-designed energy conversion systems, which seldom exceed 5% to 10% efficiency. Anaerobic Glycolysis: Generating ATP without Oxygen Up to this point we have been describing aerobic cellular respiration. We will now consider how animals generate ATP without oxygen, that is, anaerobically. Under anaerobic conditions, glucose and other 6-carbon sugars are first broken down stepwise to a pair of 3- carbon pyruvic acid molecules, yielding two molecules of ATP and four atoms of hydrogen (four reducing equivalents, represented by 2 NADH + H +). In the absence of molecular oxygen, further oxidation of pyruvic acid cannot occur because the Krebs cycle and electron transport chain cannot operate and cannot, therefore, provide a mechanism for reoxidizing the NADH produced in glycolysis. The problem is neatly solved in most animal cells by reducing pyruvic acid to lactic acid (Figure 4-16). Pyruvic acid becomes the final electron acceptor and lactic acid the end product of anaerobic glycolysis. This frees the hydrogen-bound carrier to recycle and pick up more H+. In alcoholic fermentation (as in yeast, for example) the steps are identical to glycolysis down to pyruvic acid. One of its carbons is then released as carbon dioxide, and the resulting 2-carbon compound is reduced to ethanol, thus regenerating the NAD. Anaerobic glycolysis is only one-eighteenth as efficient as complete oxidation of glucose to carbon dioxide and water, but its key virtue is that it provides some high-energy phosphate in situations in which oxygen is absent or in short supply. Many microorganisms live in places where oxygen is severely depleted, such as waterlogged soil, in mud of lake or sea bottom, or within a decaying carcass. Vertebrate skeletal muscle may rely heavily on glycolysis during short bursts of activity when contraction is so rapid and powerful that oxygen delivery to tissues is not sufficient to supply energy demands by oxidative phosphorylation alone. At such times an animal has no choice but to supplement oxidative phosphorylation with anaerobic glycolysis. Intense activity is followed by a period of increased oxygen consumption as lactic acid diffuses from muscle to the liver where it is metabolized. Because oxygen consumption increases following heavy activity, the animal is said to have acquired an oxygen debt during activity, which is repaid when activity, ceases and accumulated lactic acid is metabolized. Some animals rely heavily on anaerobic glycolysis during normal activities. For example, diving birds and mammals fall back on glycolysis almost entirely to give them the energy needed to sustain long dives. Salmon would never reach their spawning grounds were it not for anaerobic glycolysis providing almost all of the ATP used in the powerful muscular bursts needed to carry them up rapids and falls. Many parasitic animals have dispensed with oxidative phosphorylation entirely at some stages of their life cycles. They secrete relatively reduced end products of their energy metabolism, such as succinic acid, acetic acid, and propionic acid. These compounds are produced in mitochondrial reactions that derive several more molecules of ATP than does the cycle from glycolysis to lactic acid, although such sequences are still far less efficient than the classical electron transport chain. Assessment Activity This is a major activity that requires you to do the following quiz PLEASE REFER TO THE CLASSWORK IN GOOGLE CLASSROOM. MAKE SURE TO HAND IN OR SUBMIT AS DONE. Reminder. ! Before you proceed, go back and complete the Assessment above. Additional Learning Resources To learn more about the topic, please refer to the following: Additional references will be posted in the Google classroom. Please be updated. Reflecting on your Learning After engaging in all learning and assessment activities, reflect on your learning. a. Which of the seven learning outcomes presented in have you achieved? b. What are the things that are not clear to you? c. Be honest with your answers. d. Write your learning realizations on the space provided or in your Weekly journal. ______________________________________________________________________________ ______________________________________________________________________________ ______________________________________________________________________________ ______________________________________________________________________________ ______________________________________________________________________________ ______________________________________________________________________________