Neuron Types & Neuroplasticity
Document Details
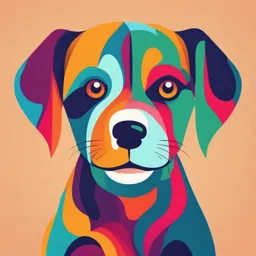
Uploaded by PreferableOrangeTree4894
Tags
Summary
This document provides an overview of different types of neurons, their structure and function. It also discusses neuroplasticity, which is the brain's ability to reorganize and form new neural connections in response to experience, learning, and environmental changes. The document further covers the process of neuroplasticity after brain injury and the key components of rehabilitation.
Full Transcript
1. Multipolar Neuron Structure: One axon and two or more dendrites Most common neuron type in the central nervous system (CNS) Key Components: Soma (cell body): Processes and integrates information Dendrites: Receive signals from other neurons Axon: Conducts impul...
1. Multipolar Neuron Structure: One axon and two or more dendrites Most common neuron type in the central nervous system (CNS) Key Components: Soma (cell body): Processes and integrates information Dendrites: Receive signals from other neurons Axon: Conducts impulses away from the soma Location: Brain Spinal cord Motor neurons Function: Mainly responsible for motor output and interneuronal communication Allows for complex signal integration due to multiple dendrites 2. Bipolar Neuron Structure: One axon and one dendrite emerging from opposite poles of the soma Less common and highly specialized Key Components: Soma: Positioned between axon and dendrite Dendrite: Receives sensory input Axon: Transmits signal to the CNS Location: Retina (eye) Olfactory epithelium (nose) Cochlea and vestibular system (inner ear) Function: Sensory functions related to vision, smell, hearing, and balance Transmits specific sensory input with high fidelity 3. Pseudounipolar Neuron Structure: A single process extends from the soma and bifurcates into two branches: a peripheral process and a central process Originates developmentally from bipolar neurons Key Components: Peripheral process: Functions like a dendrite and receives sensory input Central process: Acts like an axon, transmitting signals to the CNS Location: Dorsal root ganglia Sensory ganglia of cranial nerves Function: Transmits general sensory information such as touch, pressure, pain, and temperature Enables rapid and direct relay of signals to the CNS 4. Unipolar Neuron Structure: A single process emerges from the soma, with no clear distinction between axon and dendrite Rare in vertebrates, more common in invertebrates Key Components: Single process that may function in both receiving and transmitting signals Location: Common in invertebrate nervous systems Found during embryonic stages or in some autonomic pathways in vertebrates Function: Facilitates basic signal conduction Often involved in simpler, reflex-type responses Quick Comparison Table Neuron Type Number of Main Function Primary Location Processes Multipolar One axon, multiple Motor and integration Brain, spinal cord, motor dendrites neurons Bipolar One axon, one Special sensory (e.g., Retina, olfactory dendrite vision, smell) epithelium, ear Pseudounipola One bifurcating General sensory Dorsal root ganglia, cranial r process nerve ganglia Unipolar One process Basic signal conduction Invertebrates, embryonic (undivided) stages Structure of a Neuron A neuron is a specialized cell designed to transmit electrical signals throughout the nervous system. Its basic structure includes: Dendrites: These are short, branching extensions from the cell body. They receive chemical signals from other neurons and carry them toward the soma. Cell body (Soma): This contains the nucleus and is the main metabolic center of the neuron. It integrates incoming signals from the dendrites. Axon: A long projection that carries electrical impulses away from the soma and toward the axon terminals. Axon Terminals: These are the endpoints of the axon. They release neurotransmitters into the synaptic cleft to communicate with the next cell. Myelin Sheath: A fatty layer that wraps around the axon in segments. It insulates the axon and increases the speed of signal transmission. Nodes of Ranvier: Gaps between myelin segments where the axon membrane is exposed. These allow the action potential to jump from node to node for faster conduction. Synaptic Cleft and Signal Transmission The synaptic cleft is the tiny gap between two neurons—specifically between the axon terminal of the presynaptic neuron and the dendrite or soma of the postsynaptic neuron. Signal transmission works like this: An external or internal stimulus triggers an action potential in the presynaptic neuron. The action potential travels from dendrites → soma → axon → axon terminals. When the impulse reaches the terminals, neurotransmitters are released into the synaptic cleft. These neurotransmitters bind to receptors on the postsynaptic neuron, initiating a response. Generation of an Action Potential Neurons use action potentials (APs) to send electrical signals. An AP is an electrochemical event measured in millivolts (mV). Ion Distribution at Rest: Outside the cell: Higher concentration of calcium (Ca²⁺), chloride (Cl⁻), and sodium (Na⁺). Inside the cell: Higher concentration of potassium (K⁺). Resting membrane potential: About -70 mV (inside is more negative than outside). Step-by-Step Process: 1. Resting Potential: The neuron sits at around -70 mV, maintained by ion channels and the sodium-potassium pump. 2. Stimulus and Threshold: Neurotransmitters bind to receptors on the postsynaptic cell. If excitatory signals depolarize the cell to -55 mV (threshold), an action potential is triggered. Subthreshold stimuli (less than -55 mV) do not cause an AP (this is called failed initiation). 3. Depolarization: At -55 mV, voltage-gated sodium (Na⁺) channels open, and Na⁺ rapidly flows into the neuron. The inside becomes more positive, reaching up to about +30 mV. 4. Repolarization: Na⁺ channels close, and voltage-gated potassium (K⁺) channels open. K⁺ flows out of the cell, making the inside more negative again. 5. Hyperpolarization (Refractory Period): Too much K⁺ leaves the cell, causing the membrane potential to dip below -70 mV, down to about -90 mV. During this time, the neuron cannot initiate another AP, which ensures one-way transmission and recovery. 6. Return to Resting Potential: K⁺ channels close, and the Na⁺/K⁺ pump restores ion balance (3 Na⁺ out, 2 K⁺ in), returning the cell to -70 mV. Synaptic Transmission Steps Recap: 1. Action potential reaches the presynaptic axon terminal. 2. Voltage-gated Ca²⁺ channels open, allowing calcium to enter. 3. Calcium causes synaptic vesicles to fuse with the membrane. 4. Neurotransmitters are released into the synaptic cleft. (acetylcholine) 5. Neurotransmitters bind to receptors on the postsynaptic membrane. 6. This opens ion channels (e.g., Na⁺ or Cl⁻ channels). ○ If Na⁺ enters: the postsynaptic cell depolarizes, moving closer to threshold. ○ If Cl⁻ enters: the cell hyperpolarizes, moving further from threshold. Key Concepts of Action Potentials All-or-None Law: If the threshold is reached, the AP always fires at full strength. If not, it doesn’t fire at all. Subthreshold Stimuli: These do not reach the -55 mV threshold and cannot trigger an AP. Suprathreshold Stimuli: These exceed the threshold and can cause APs at higher frequencies, especially after the refractory period ends. Speed of Action Potential Propagation Several factors affect how fast an action potential travels: 1. Axon Diameter: Larger-diameter axons conduct impulses faster due to less resistance to ion flow. 2. Myelin Sheath: Myelin insulates the axon and speeds up the transmission by forcing the AP to jump between the nodes of Ranvier—a process called saltatory conduction. 3. Integrity of Myelin: Myelin must be intact to maintain speed. In diseases like Multiple Sclerosis, myelin is damaged, leading to slower and less efficient AP conduction. Neurotransmitters and Postsynaptic Potentials When neurotransmitters are released into the synaptic cleft, they bind to specific receptors on the postsynaptic membrane. The type of ion channel they open determines the type of postsynaptic potential that occurs. 1. Excitatory Postsynaptic Potential (EPSP) Cause: Influx of positive ions, usually sodium (Na⁺) Effect: ○ Makes the inside of the postsynaptic neuron less negative ○ Brings the membrane potential closer to the threshold for firing an action potential (e.g., from -70 mV to -55 mV) Neurotransmitters involved: ○ Common examples: Glutamate, acetylcholine Result: Increased likelihood of generating an action potential 2. Inhibitory Postsynaptic Potential (IPSP) Cause: Influx of negative ions, typically chloride (Cl⁻) (or sometimes efflux of K⁺, which is also inhibitory) Effect: ○ Makes the inside of the postsynaptic neuron more negative ○ Moves the membrane potential further from the threshold Neurotransmitters involved: ○ Common examples: GABA, glycine Result: Decreased likelihood of an action potential being triggered Summary Type Ion Resulting Charge Effect on Common Movement Change Neuron Neurotransmitters EPS Influx of Na⁺ Less negative Increases Glutamate, P (depolarization) excitability Acetylcholine IPSP Influx of Cl⁻ More negative Decreases GABA, Glycine (hyperpolarization) excitability What is a Seizure? A seizure is a sudden, uncontrolled surge of electrical activity in the brain. It occurs when multiple neurons fire simultaneously in a hyper-synchronized way. Seizures are typically classified into focal and generalized types, depending on where in the brain they begin. Focal Seizures (also called Partial Seizures) Definition: Start in a specific, localized area of one cerebral hemisphere. Key Characteristics: May affect movement, sensation, emotion, or autonomic functions depending on the brain region involved. Consciousness can be preserved or impaired: ○ Focal aware seizure (formerly simple partial): Awareness is intact. ○ Focal impaired awareness seizure (formerly complex partial): Awareness is altered or lost. May present with: ○ Muscle jerking in one body part (e.g., hand, face) ○ Sensory disturbances (tingling, visual changes, unusual smells or tastes) ○ Emotional or psychic symptoms (e.g., fear, déjà vu) Can evolve into secondary generalized seizures. Primary Generalized Seizures Definition: Originate simultaneously across both hemispheres of the brain, without a localized starting point. Key Characteristics: Consciousness is always impaired to some degree. Often associated with genetic or idiopathic epilepsy syndromes. EEG shows generalized discharges from the onset. No warning or aura since the whole brain is affected at once. Types of Primary Generalized Seizures: Absence seizures (formerly "petit mal"): Brief lapses in consciousness (usually 5–15 seconds), often with eye blinking or lip smacking. Tonic-clonic seizures (formerly "grand mal"): Loss of consciousness followed by stiffening (tonic phase) and rhythmic jerking (clonic phase). Tonic seizures: Sudden increase in muscle tone, often causing a fall. Clonic seizures: Repetitive, rhythmic muscle jerks. Myoclonic seizures: Sudden, brief muscle jerks without loss of consciousness. Atonic seizures: Sudden loss of muscle tone, leading to collapse or head drop. Secondary Generalized Seizures Definition: Begin as a focal seizure in one area of the brain, then spread to involve both hemispheres, becoming generalized. Key Characteristics: Start with focal symptoms (e.g., aura, localized motor/sensory signs). Progress into a generalized seizure, typically tonic-clonic in nature. Often seen in people with structural brain abnormalities or focal epilepsy. Summary Table Seizure Type Origin Consciousness Features Focal Seizure One hemisphere May be preserved Localized symptoms, aura, (localized focus) or impaired can spread to become generalized Primary Both hemispheres Always impaired Immediate widespread Generalized simultaneously symptoms; no aura; EEG Seizure shows generalized onset Secondary Begins focal, Initially preserved Starts focal, becomes Generalized spreads bilaterally or impaired tonic-clonic; often with aura Seizure Spontaneous Biological Recovery Definition: Spontaneous biological recovery refers to the brain’s natural, short-term recovery process following injury (such as stroke or trauma), without the aid of external interventions like rehabilitation or medication. Timeline: Begins within days to weeks after the injury May continue up to six months in some cases This recovery is largely due to the resolution of acute physiological responses to the injury rather than true functional reorganization. Short-Term Mechanisms of Spontaneous Recovery 1. Resolution of Diaschisis Definition: Diaschisis is a temporary loss of function in healthy brain regions that are functionally connected to the damaged area via neural pathways. Cause: Hypoperfusion: Reduced blood flow to connected areas Hypometabolism: Decreased energy metabolism in those areas Effect: Although these regions are structurally intact, they become functionally suppressed due to their connections with the injured site. As blood flow and metabolism improve, these areas may gradually regain function. 2. Edema (Brain Swelling) Edema refers to the accumulation of excess fluid in brain tissue following injury. It contributes to temporary loss of function by compressing neurons and disrupting normal cell signaling. There are two main types: a) Cytotoxic Edema (Cellular Edema) Cause: Malfunction of the sodium-potassium pump in neurons leads to sodium (Na⁺) accumulating inside cells. Mechanism: Water follows Na⁺ into the cells via osmosis → cell swelling Outcomes: If reversible: cells recover If prolonged: can result in cell death b) Vasogenic Edema Cause: Damage to the blood-brain barrier allows proteins and fluid to leak into the extracellular space Mechanism and Effects: Extracellular fluid buildup compresses nearby tissues Disrupts synaptic function and neurotransmitter activity Can impair signal conduction between neurons Fluid may draw water out of cells (via osmosis), potentially shrinking cells and further disturbing function 3. Denervation Supersensitivity Definition: A process in which postsynaptic neurons become hypersensitive due to reduced input from damaged presynaptic neurons. Mechanism: Injury reduces presynaptic neurotransmitter release In response, postsynaptic neurons upregulate receptors or become more sensitive to the remaining neurotransmitters Effects: Increased excitability of the postsynaptic neuron May cause hyperactive reflexes and increased muscle tone If not normalized, may lead to long-term maladaptive plasticity (e.g., spasticity or rigidity) Summary Mechanism Key Cause Impact on Recovery Resolution of Hypoperfusion / Restores function to regions temporarily Diaschisis hypometabolism in suppressed after injury connected areas Cytotoxic Edema Na⁺ pump failure → Swollen neurons may recover or die, intracellular fluid depending on severity accumulation Vasogenic Edema Leaky blood-brain barrier → Compresses brain tissue, impairs extracellular fluid synapses and signaling Denervation Reduced input → increased Heightens excitability; may lead to Supersensitivity postsynaptic sensitivity functional compensation or pathological hyperactivity Neurogenesis and Cell Proliferation Neurogenesis is the process by which new neural cells (neurons and glial cells) are created through cell proliferation — the division of neural stem cells. 1. Where Does Neurogenesis Occur? Neurogenesis is most active during embryonic and early childhood development, but also occurs to a lesser extent throughout adulthood in specific regions of the brain: Hippocampus – involved in learning and memory Amygdala – involved in emotional regulation Lateral walls of the lateral ventricles – source of neural stem cells in the adult brain 2. How Do Neural Cells Form? Neural stem cells (NSCs) are undifferentiated cells with the potential to divide and form all major cell types in the nervous system. When NSCs divide, they can become: A. Neuronal Progenitor Cells Mature into neurons Neurons are the functional cells of the nervous system Responsible for sending and receiving electrochemical signals Support sensory, motor, and cognitive-perceptual functions in the CNS and PNS B. Glial Progenitor Cells Mature into different types of glial cells, which support and protect neurons 3. Types of Glial Cells (Formed Through Proliferation) A. Microglia Act as the primary immune defense in the CNS Remove pathogens and cellular debris Involved in synaptic and dendritic pruning — removing unnecessary neural connections B. Macroglia 1. Astrocytes ○ Provide metabolic support to neurons ○ Help maintain the blood-brain barrier ○ Regulate neurotransmitter levels in the synapse ○ Release signaling molecules that influence synaptic transmission 2. Oligodendrocytes (CNS) ○ Produce myelin to insulate axons ○ Enhance the speed of electrical signal transmission ○ Equivalent to Schwann cells in the PNS 3. Ependymal Cells ○ Line the ventricles of the brain ○ Produce and help circulate cerebrospinal fluid (CSF) 4. Radial Glia ○ Act as progenitor cells during development ○ Guide neuronal migration in the developing brain ○ In adults, contribute to ongoing neurogenesis in certain brain regions Summary of Neurogenesis and Cell Types Cell Type Origin Function Neuron From neuronal progenitor Transmit electrical impulses; key for sensory, cells motor, cognition Astrocyte From glial progenitor cells Support neurons, regulate synapses, form BBB Oligodendrocyt From glial progenitor cells Myelinate CNS axons e Ependymal Cell From glial progenitor cells Produce cerebrospinal fluid Radial Glia From neural stem cells Act as scaffolding and stem cells during development Microglia Originate from yolk sac Immune defense and synaptic pruning in the (not NSCs) CNS Neuroplasticity Overview Neuroplasticity is the brain’s ability to reorganize and form new neural connections in response to experience, learning, and environmental changes. It plays a crucial role in behavioral learning, adaptation to new environments, and recovery from brain injury. As stated by Kleim & Jones (2008): "Neuroplasticity is the mechanism by which the brain encodes experience and learns new behaviors. It is also the mechanism by which the damaged brain relearns lost behavior in response to rehabilitation." This means the brain is dynamic and constantly evolving based on experiences or injuries. Neuroplasticity can be beneficial (helpful in learning and recovery) or maladaptive (leading to the reinforcement of undesirable behaviors). 1. Types of Neuroplasticity Neuroplasticity can be categorized into chemical, structural, and functional changes: A. Chemical Plasticity (Synaptic Plasticity) Synaptic plasticity refers to changes in the efficiency of synaptic transmission, primarily through changes in the synapses (the communication points between neurons). Mechanisms of Synaptic Plasticity: Increased or decreased frequency of action potentials alters synaptic activity and the strength of synaptic connections. This affects: ○ The efficiency of ion exchange across the synapse (e.g., Ca²⁺, Cl⁻, Na⁺, K⁺). ○ The number of neurotransmitters released by the pre-synaptic neuron. ○ The number of receptors on the post-synaptic neuron. Outcome: Synaptic plasticity allows the brain to strengthen or weaken neural connections, facilitating learning, memory formation, and adaptation to new experiences. Example: Long-Term Potentiation (LTP): Increased synaptic strength, a process related to memory and learning. Long-Term Depression (LTD): Decreased synaptic strength, related to forgetting or unlearning. B. Structural Plasticity Structural plasticity refers to physical changes in the brain's structure, particularly in the dendrites and axons of neurons. These changes are often the result of learning or brain injury and involve alterations in the connections between neurons. i) Dendritic Plasticity Dendrites are the branching extensions of neurons that receive signals from other neurons. Dendritic plasticity involves changes in the number and structure of dendrites and dendritic spines (small protrusions on dendrites where synapses form). Key Processes: 1. Dendritic Sprouting/Arborization: ○ The growth of new dendrites and spines to form new synaptic connections (synaptogenesis). ○ This is beneficial for learning and memory. 2. Dendritic Pruning: ○ The elimination of weak or unused dendritic connections to make the neural network more efficient. ○ This process refines the brain’s circuits. ii) Axonal Plasticity Axons are long, threadlike extensions of neurons that transmit electrical signals to other neurons or muscles. Axonal plasticity involves the rerouting or sprouting of new axons to innervate new neurons or areas that were previously not connected. Key Processes: 1. Axonal Sprouting: ○ The formation of new axonal branches from the existing axon. ○ This can help restore function after injury by forming new connections to previously inactive neurons. 2. Axonal Rerouting: ○ Redirecting axons to form connections with different neurons, potentially compensating for damaged pathways. C. Functional Plasticity (Topographical Re-Mapping) Functional plasticity refers to the reorganization of brain regions in response to changes in the environment or following brain injury. It involves the shift of neural functions from damaged to undamaged brain areas. Topographical Re-mapping: This process involves the brain's ability to shift the function of neural circuits to other regions in response to damage or learning. Experience-dependent plasticity is central here—neurons that fire together, wire together. This means that experiences and behaviors shape the connections between neurons. Merzenich et al. (1983) – Reorganization of Functional Areas After Injury: Following brain injury (such as a stroke), areas of the brain that were previously responsible for certain functions (e.g., movement, sensation) can be re-mapped to new areas. This phenomenon is important in rehabilitation. For example, after losing the ability to move a limb, neural reorganization can help other regions of the brain take over the function. "Neurons that fire together, wire together": This famous phrase by Donald Hebb explains that repeated, coordinated neural activity strengthens the synaptic connection between neurons. This is the basis for learning, memory, and adaptive changes after injury. Summary of Neuroplasticity Types: Type of Key Features Examples/Outcomes Neuroplasticity Chemical (Synaptic Changes in synaptic Strengthening or weakening of neural Plasticity) strength and efficiency connections (LTP, LTD), memory formation Structural (Dendritic Changes in the number Dendritic sprouting (new synaptic Plasticity) and structure of connections), pruning (eliminating weak dendrites connections) Structural (Axonal Rerouting or sprouting Restoration of function after injury, new Plasticity) of axons pathways formed Functional Reorganization of brain Brain regions shift functions to (Topographical functions after damage compensate for damage (e.g., motor cortex Re-mapping) reorganization after stroke) Neuroplasticity: A Dual Mechanism Beneficial Changes: Neuroplasticity allows the brain to adapt to new experiences, learn new skills, and recover from injury. Maladaptive Changes: Neuroplasticity can also reinforce unwanted behaviors (e.g., chronic pain, addiction) or result in maladaptive neural circuits. Short-Term Potentiation (STP) Short-Term Potentiation (STP) refers to the temporary strengthening of synaptic connections between neurons, which leads to an increase in action potential (AP) firing. This phenomenon is critical in understanding how synaptic efficiency improves for short durations and plays a role in memory and learning. Process: ○ STP happens at the chemical level involving neurotransmitters and receptors. ○ It involves a temporal enhancement of synaptic connections, meaning that the synapses become more efficient at transmitting signals for a brief period of time. Duration: ○ STP typically lasts around 20-40 minutes, but the exact duration depends on the frequency and timing of action potentials (APs) firing in the area. ○ STP may be part of a continuum that leads to Long-Term Potentiation (LTP), which lasts longer. Connection to Memory: ○ STP is thought to play a role in short-term and working memory. The efficiency of synaptic connections during STP allows for quick processing of information, but it doesn’t last long enough to become long-term memory. ○ Studies (Bliss et al., 2018; Lisman, 2017) suggest that STP could be foundational in learning processes, allowing the brain to initially adapt before consolidating memories for the long term. Long-Term Potentiation (LTP) Long-Term Potentiation (LTP) is the process through which repeated stimulation of synapses leads to long-lasting changes in synaptic strength and structure. These changes are crucial for memory consolidation, learning, and skill acquisition. Process: ○ LTP is the result of the persistent firing and strengthening of synapses during STP, which causes structural and functional changes in the brain. ○ These changes include synaptic, dendritic, and axonal plasticity that remain for extended periods, supporting sustained memory and learning. Key Features of LTP: ○ Persistent synaptic strengthening: LTP occurs when repetitive, high-frequency stimulation of synapses causes long-lasting changes in their strength and the connections between neurons. ○ These structural changes are responsible for sustained memories, learning, and the acquisition of new skills. Rehabilitation Connection: ○ In the context of motor rehabilitation, such as with the upper extremity (UE) after stroke or injury, repetitive, structured task-performance (with restraint of the unaffected UE) leads to neural reorganization. ○ This process stimulates synaptic connections, dendritic sprouting, and axonal rerouting in the motor cortex, which supports the recovery of motor function and minimizes learned non-use (when the unaffected side compensates too much). ○ Key Requirements for Effective Rehabilitation: Patients should show some level of active wrist extension and finger/thumb movement. The damage to the brain should not be too extensive, and some undamaged brain regions must still be available for reorganization. Intensive intervention (4-7 hours per day for 10-14 days) is typically required for promoting neuroplastic changes. Neural Reorganization After Injury Neural changes occur both in the healthy brain and after brain injury. This reorganization is directly influenced by experiential and behavioral events, meaning that experiences and behaviors drive the brain's capacity to adapt. Key Reorganization Processes: Synaptic Stimulation and Plasticity: Repeated stimulation strengthens synaptic connections, leading to more efficient communication between neurons. This is essential in learning new skills or recovering lost abilities after brain injury. Dendritic Sprouting (Synaptogenesis) vs. Pruning: ○ Synaptogenesis: The formation of new synapses as dendrites sprout to form new connections, which is essential for learning and recovery. ○ Pruning: The process of eliminating unnecessary or unused synapses, optimizing neural circuits. Axonal Sprouting and Re-routing: ○ Damaged axons may grow new branches (sprouting) to re-establish connections with neurons in the affected region, or they may re-route to innervate new areas of the brain to compensate for lost functions. Topographical Re-mapping: Following injury, the brain can re-map functions from damaged areas to adjacent, undamaged areas. This reorganization allows for the recovery of lost functions, such as movement or sensation. Neurogenesis: The formation of new neurons in specific regions of the brain (such as the hippocampus) helps with recovery and memory formation. This process is more prominent in critical periods of development but continues at a lower rate in adulthood. Changes in Vasculature and Glia: ○ Vascular changes support new neural growth by improving blood flow and oxygen delivery to the brain. ○ Glial cells (such as astrocytes, microglia, and oligodendrocytes) assist in maintaining the health of neurons, providing support, and cleaning up after injury. Factors Affecting Recovery 1. Age ○ Mature Brain Areas: If injury occurs in well-developed, functionally mature areas, the recovery outcome is similar for both adults and children. ○ Mature Brain Adjacent to Immature Areas: If a mature brain area is near an immature area, the immature region may take over the function of the injured area. ○ Immature Areas Without Compensation: If the injured area is not fully developed and no other area can assume its function during maturation, recovery can vary. The brain may recover fully or appear recovered early on, but issues might emerge later in adulthood. 2. Lesion ○ Lesion Size: Smaller lesions tend to result in better recovery outcomes compared to larger lesions. ○ Development of Lesion: Slow-developing lesions cause less functional loss than rapid-onset lesions. ○ Depth of Lesion: Lesions that affect deeper brain structures typically result in more severe impairments than those that are more superficial. ○ Lesion Area: Areas with collateral circulation may support better recovery, as surrounding tissue can help compensate for the damage. 3. Duration and Severity of Ischemic Attack ○ The longer and more severe the ischemic event, the greater the potential for brain damage, impacting recovery. Early intervention can help mitigate long-term effects. 4. Environment ○ Enriched Environments: Stimulation from the environment (e.g., physical, social, and cognitive activities) accelerates recovery and neuroplastic changes. 5. Time ○ Window for Treatment: Early intervention is key to recovery, but there’s a balance. Treatment should start soon after the injury but not be rushed too quickly. The timing can influence the extent of neuroplastic changes and recovery. 6. Other Factors ○ Social Support: Emotional, social, and financial support plays a significant role in an individual’s ability to recover and engage in rehabilitation. ○ Personal Factors: Cognitive, emotional, and physical barriers can affect the client’s ability to participate in recovery activities. 10 Principles of Neuroplasticity 1. Use It or Lose It: Neurons that are not used regularly will degrade over time, which can impair function. 2. Use It and Improve It: Regularly engaging brain regions can improve their function, promoting recovery or enhancing performance. 3. Specificity: Recovery is most effective when training or rehabilitation is specific to the task or function that needs to be restored. 4. Repetition Matters: Repeated activity helps reinforce neural connections, leading to more effective recovery. 5. Intensity Matters: Higher intensity interventions lead to more significant changes in neuroplasticity and recovery. 6. Time Matters: Neuroplastic changes need to occur within a specific time frame for the most effective results, with early intervention yielding the best outcomes. 7. Salience Matters: The rehabilitation task must be meaningful or relevant to the individual for optimal recovery, ensuring that the experience drives neural changes. 8. Age Matters: Younger brains tend to recover more readily due to their greater plasticity, though older brains are still capable of significant recovery with appropriate interventions. 9. Transference: Skills and experiences learned in one area of the brain can transfer to other related areas, facilitating broader recovery. 10.Interference: Interventions or experiences that interfere with normal brain activity or neuroplasticity can impede recovery.