Cellular Mechanisms of Development - Chapter 10: Pregnancy, Development, and Aging
Document Details
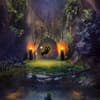
Uploaded by iiScholar
Arizona State University
Tags
Summary
This document covers the cellular processes and concepts central to developmental biology in Chapter 10. Key aspects of the document include stem cell potency and specialization. The document focuses on cell division and self-renewal, cell specification and determination, and gene regulation.
Full Transcript
**Cellular Mechanisms of Development** Introduction Mammalian development begins with the zygote, a single cell that gives rise to all tissue types and organs in the mature organism. The organization and transformation of cells during the developmental period are carried out by a complex array of...
**Cellular Mechanisms of Development** Introduction Mammalian development begins with the zygote, a single cell that gives rise to all tissue types and organs in the mature organism. The organization and transformation of cells during the developmental period are carried out by a complex array of cellular mechanisms that depend on the precise coordination of signal and response (ie, development is highly regulated in both space and time). This lesson provides an overview of some of the cellular and molecular mechanisms that can be used to execute a developmental plan. Mechanisms such as asymmetrical cell division, cell signaling and migration, differential gene expression, and programmed cell death act synergistically to drive the proper patterning and growth of the embryo. 10.3.01 Cell Potency and Specialization A single-celled zygote has the potential to give rise to every cell type (ie, both embryonic and extraembryonic) and is thus considered **totipotent** (Figure 10.10). Because of asymmetrical cell division during embryonic cleavage, the fate of specific embryonic blastomeres becomes progressively restricted beyond the 8-cell stage. As an embryo progresses toward blastulation, individual blastomeres are restricted to either embryonic or extraembryonic cell types; therefore, the blastomeres are no longer totipotent. Instead, these cells are considered **pluripotent** (ie, able to give rise to either embryonic or extraembryonic cell types but not both). For example, as the embryo approaches the blastocyst stage, trophoblast cells are already restricted to extraembryonic cell fates (eg, precursors to the placenta) and are no longer able to contribute to the embryo itself (Figure 10.10). As development continues, the fate of individual embryonic cells becomes even more restricted. At neural tube closure, the cells of the neural tube are **multipotent**, or able to generate multiple cell types within a restricted population or lineage (eg, ectodermal cells are restricted to neural, epidermal, and neural crest cell fates). Continued development may yield further restriction of multipotent cells or **unipotent** cells (ie, generate only one cell type). Through [differential gene expression](javascript:void(0)), **terminal differentiation** of these cells leads to unique cell types with characteristic biochemical signatures, structures, and functions. Chapter 10: Pregnancy, Development, and Aging 349 **Figure 10.10** Cell potency. **Stem cells** retain their potency and ability to divide but may generate daughter cells that terminally differentiate into specific cell types. Depending on the source, stem cells can give rise to many cell types (eg, pluripotent embryonic stem cells of the inner cell mass) or only a few (eg, multipotent [hematopoietic](javascript:void(0)) [stem cells](javascript:void(0))). Although stem cells are usually discussed in the context of embryonic development, it is important to note that stem cell populations also exist in many adult tissues (eg, bone marrow, brain). Some stem cell populations can **self-renew**. As a result of asymmetrical cell division, one daughter cell may terminally differentiate into a specific cell type, whereas the other retains stem cell properties that maintain the progenitor cell pool (Figure 10.11). For example, during early spermatogenesis, unipotent spermatogonial stem cells generate daughter cells that either differentiate into primary spermatocytes or maintain the spermatogonial stem cell population so that additional spermatozoa can be generated continually throughout life (see Concept 9.2.02). A diagram of a cell AI-generated content may be incorrect. Chapter 10: Pregnancy, Development, and Aging 350 If stem cell division is symmetrical, both daughter cells may retain stem cell properties in some instances, thereby enlarging the stem cell population. In other instances, both daughter cells derived from the stem cell may terminally differentiate, effectively depleting the stem cell population over time (Figure 10.11). **Figure 10.11** Stem cell division and self-renewal. The process by which cells become committed to a specific cell fate occurs in stages. Totipotent cells are said to be unspecified. **Cell specification** begins very early in development when a cell becomes capable of differentiating into a particular cell type on its own in a neutral environment. However, the fate of a specified cell is not fixed and may be changed by external cues. For example, a cell specified as mesodermal may retain the potential to become a neuron if placed in conditions which induce neuronal development. A cell is said to be **determined** when it is tied to a specific fate, even when placed in an alternate environment (ie, the cell\'s developmental fate is *not* altered by external cues). For example, once a mesodermal cell is determined as a muscle cell precursor, that mesodermal cell cannot become a neuron, even when placed in conditions which induce neuronal development. Once determined, progenitor cells are induced to undergo terminal differentiation, developing specialized structures and functions. In some cases (eg, neuronal progenitor cells), terminally differentiating cells may leave the [cell cycle](javascript:void(0)) and do not divide again. 10.3.02 Gene Regulation in Development A fundamental question in development centers on how differences arise in seemingly equivalent (ie, totipotent) embryonic cells. Although the full mechanism is unknown, the progressive determination and differentiation of unique cell types are directed by differential gene expression resulting from a combination of cell **intrinsic** (internal) and **extrinsic** (external) **factors**. Early embryogenesis is driven largely by intrinsic factors, with later patterning involving extrinsic factors, resulting in progressive cell fate restriction, as summarized in Table 10.2.  Chapter 10: Pregnancy, Development, and Aging 351 **Table 10.2** Cell intrinsic and extrinsic factors in early embryogenesis. In the early cleavage stage of embryogenesis, cell division is rapid and the embryo is dependent on oocyte stores of mRNA and proteins. The embryonic genome becomes active beginning at the 4- to 8-cell stage, but oocyte contributions persist through the blastocyst stage. The embryonic genome must undergo extensive epigenetic remodeling before genome activation. This remodeling allows [transcription](javascript:void(0)) [factors](javascript:void(0)) better access to [chromatin](javascript:void(0)), which is necessary for the proper regulation of embryonic gene expression. During remodeling, [cytosine nucleotides](javascript:void(0)) may be [methylated](javascript:void(0)), which generally has a silencing effect on gene expression. Most genomic DNA is demethylated shortly after fertilization; however, for certain genes, the maternal and paternal methylation patterns remain intact, and alleles are not functionally equivalent. Therefore, methyl group retention on specific alleles leads to parent-specific gene expression in offspring, a phenomenon known as **genomic imprinting** (Figure 10.12). When the paternal allele is imprinted (ie, methylated), gene expression occurs only from the maternal allele and vice versa. A chart of a cell extrinsic factor AI-generated content may be incorrect. Chapter 10: Pregnancy, Development, and Aging 352 **Figure 10.12** Genomic imprinting. Because of differences in primary sex determination, individuals with two X chromosomes have twice as many copies of X chromosome genes than XY individuals. This phenomenon has important implications in terms of X-linked inheritance (discussed in Concept 7.3.02). XX individuals compensate for this disparity via **X chromosome inactivation** (**X inactivation**) in early embryonic development. During X inactivation, one X chromosome in each cell becomes inactivated due to expression of the *XIST* (X-inactive specific transcript) gene. *XIST* expression initiates epigenetic X chromosome modification, resulting in extensive chromatin remodeling, DNA methylation, and gene silencing (see Concept 2.4.02). This causes the X chromosome expressing *XIST* to condense, forming a compact structure known as a **Barr body**. X chromosome inactivation occurs in each embryonic cell on a random basis. Therefore, in any given cell from an XX individual, the active X chromosome may be of paternal or maternal origin. If an XX individual is [heterozygous](javascript:void(0)) for an [X-linked](javascript:void(0)) trait, roughly half of the individual\'s cells express the maternal allele, and the other half express the paternal allele (Figure 10.13).  Chapter 10: Pregnancy, Development, and Aging 353 **Figure 10.13** X chromosome inactivation. As discussed in Concept 2.4.03, regulatory elements found outside of DNA coding regions contain enhancer sequences that, when bound by transcription factors, modulate gene expression levels. Tissue-specific gene expression during development arises due to unique combinations of transcription factors expressed in individual cells. Enhancer sequences are often modular, meaning that distinct enhancers associated with a single gene can regulate that gene\'s expression in different tissues and at different times during development. Only specific combinations of transcription factors bound to an enhancer allow a gene to be expressed in a particular cell type. This same gene remains unexpressed in cells that do not have the correct combination of transcription factors. For example, the transcription factor PTF1A is known to be expressed in both pancreatic and cerebellar progenitor cells during development (Figure 10.14). Two distinct regulatory enhancers controlling *PTF1A* expression exist: one that controls expression in the developing cerebellum and one that controls expression in the developing pancreas. Mutations within individual enhancers disrupt development in a tissue-specific fashion, affecting either the pancreas or cerebellum but not both (ie, a mutation in the pancreatic enhancer affects the pancreas, but not the cerebellum). Diagram of a cell with different types of cells AI-generated content may be incorrect. Chapter 10: Pregnancy, Development, and Aging 354 **Fig 10.14** Example of enhancer sequence modularity. In addition to an organism\'s genetic makeup, environmental conditions also play a role in development. Environmental cues may influence organism development by altering cell signaling and gene expression at crucial times during development. Epigenetic changes due to diet, temperature, and other environmental exposures may alter the progression of development in both small and highly impactful ways. One well-known example of environmental effects during human development involves deficiencies in dietary folate. Low maternal folate intake can affect neural tube closure in the developing embryo and is one of the leading causes of neural tube defects in humans. Although the exact mechanism is unknown, it is hypothesized that disruption of folic acid metabolism may affect DNA methylation in the developing nervous system. It is estimated that 25%-30% of neural tube defects in humans can be prevented by taking supplemental folate during pregnancy. 10.3.03 Cell Signaling and Migration Cell-cell signaling drives many developmental cellular activities (eg, division, adhesion, migration, differentiation). For example, ectodermal cells are induced to become neural plate cells in response to signal secretion by the underlying notochord (see Concept 10.2.02). **Induction** involves the cells or tissues that produce signaling molecules (ie, **inducers**) and the responding cells or tissues, which must be **competent** (ie, able to receive and respond to inductive signals). For example, a secreting cell induces differentiation only in neighboring cells that express the correct receptors and signal transduction machinery. Often, inductive interactions are reciprocal: The inducing cells trigger the secretion of new inductive signals in the neighboring cells, which then act on the original inducing cells to further refine developmental fates. For example, in [vertebrate eye](javascript:void(0)) development, the ectoderm overlying the optic vesicle (ie, developing eye) induces lens formation, and the newly formed lens cells reciprocate, instructing the optic vesicle to form the retina. Inducers are most often paracrine factors but may also be autocrine or juxtacrine signals (Figure 10.15). Secreted **paracrine** signals exert their effects on nearby cells via diffusion, whereas **autocrine** signals exert effects on the same cell from which they are secreted. **Juxtacrine** signals are cell surface ligands from one cell that interact with receptors on adjacent cells. Before circulatory system development, endocrine signaling is not prominent.  Chapter 10: Pregnancy, Development, and Aging 355 **Figure 10.15** Types of inductive cell signaling. Signaling gradients are a key feature of early embryonic development. **Morphogens** are inductive paracrine factors that diffuse from a signaling cell to form a concentration gradient within nearby tissues. The fates of receiving (ie, competent) cells within a diffusible distance of the morphogen are influenced by the concentration of morphogen to which the receiving cells are exposed (Figure 10.16). In addition to diffusion, morphogen gradients are influenced by time-dependent morphogen destruction and/or uptake into cells. Overlapping morphogen gradients may be used to establish precise patterns of gene expression and cell differentiation during development. **Figure 10.16** Morphogens influence the fates of competent cells based on morphogen concentration.  Chapter 10: Pregnancy, Development, and Aging 356 For example, morphogen concentration in the neural tube influences the differentiation of spinal neuron populations along the dorsal-ventral axis of the neural tube. Opposing concentrations of the morphogens bone morphogenetic protein (BMP), Wnt, and sonic hedgehog (Shh) in the neural tube specify dorsal and ventral cell fates (Figure 10.17). High levels of BMP and Wnt are secreted from the overlying ectoderm and most dorsal regions of the neural tube, inducing gene expression that leads to dorsal cell fates (ie, differentiation into [sensory](javascript:void(0)) [neurons](javascript:void(0))). In contrast, high levels of Shh secretion from the notochord and most ventral regions of the neural tube induce the expression of genes that lead to ventral cell fates (ie, differentiation into motor neurons). **Figure 10.17** Opposing morphogen gradients in neural tube development. Although less common than paracrine signaling, some juxtacrine signaling pathways are crucial in developmental biology. For example, the Notch pathway involves a Notch receptor protein embedded in an inducing cell membrane that interacts with a ligand (eg, Delta) embedded in the receiving cell membrane. Adjacent progenitor cells may often express both the Notch receptor and its ligand. This type of signaling may be used to induce the differentiation of one progenitor cell while keeping the other in an undifferentiated state, a process known as **lateral inhibition**. For example, in two adjacent cells, the cell in which the Notch pathway is first engaged leads to the inhibition of the Delta ligand within the same cell, thereby preventing the ligand from activating Notch in the adjacent cell. Therefore, the first cell to activate the Notch pathway undergoes differentiation, and the adjacent cell remains undifferentiated, as shown in Figure 10.18. A diagram of a brain AI-generated content may be incorrect. Chapter 10: Pregnancy, Development, and Aging 357 **Figure 10.18** Notch signaling and lateral inhibition. As development proceeds, some cells must migrate short and long distances to arrive at the appropriate locations. For example, during gastrulation, many complex cell movements and rearrangements occur to transform the embryo from a flat, two-layered disk into a complex tubular structure. Cells migrate by responding to environmental cues and surrounding cells. Because of embryonic patterning, a migrating cell encounters different environments as it moves within the embryo. Cells migrate (either individually or in groups) in response to short- and long-range signals to reach their final destination. The migration of neural crest cells is an example of cell migration during development (see Concept 10.2.02). Following neural tube closure, some cells (ie, neural crest cells) lose their adhesive junctions and separate from the epithelium in a process called **delamination**. Once delaminated, neural crest cells migrate extensively along the anterior-posterior axis, giving rise to a wide variety of different cell types, including cells of the peripheral nervous system, adrenal medulla, melanocytes, and head/neck connective tissues. Both external (eg, environmental signals) and internal factors (eg, changing patterns of adhesion proteins) drive neural crest migration. These factors guide cells via both attractive and repulsive interactions through different environments and over long distances. 10.3.04 Programmed Cell Death Just as cell proliferation is crucial during embryonic development and morphogenesis, so too is restricting the number of cells produced. Normal development often generates an excess number of cells, with surplus cells later removed via [apoptosis](javascript:void(0)) (ie, programmed cell death). During mammalian development, apoptosis occurs at several points, including during blastocyst formation, shaping of tubular structures, and separation of digits (Figure 10.19).  Chapter 10: Pregnancy, Development, and Aging 358 Correctly timed apoptosis is critical during vertebrate limb development. During development, each digit is specified by exposure to sequential signaling gradients (eg, morphogens) from the posterior interdigital tissue (ie, mesodermal tissue between each digit). After digit formation, apoptosis of the interdigital webbing is induced to separate the limb bud into distinct digits. **Figure 10.19** Apoptosis in the separation of digits. **Concept Check 10.3** Indicate whether each of the following statements about cell-cell signaling during development are true or false and why. 1.Cells in a developing tissue can respond to multiple morphogens, leading to the establishment of more complex differentiation patterns. 2.Lateral inhibition is a mechanism by which cells in a developing tissue enhance each other\'s differentiation, leading to uniform cellular identities. 3.During embryonic development, the timing of induction is critical because cells can respond to certain inductive signals only during specific developmental stages. [**Solution**](javascript:void(0)) A diagram of a limb AI-generated content may be incorrect. **Gestation** Introduction **Gestation** (ie, pregnancy) generally lasts approximately 38 weeks, from ovulation until birth. However, the gestation period is often expressed as 40 weeks when considered from the beginning of the last menstrual cycle. During gestation, many maternal changes occur to support the growing fetus and to prepare the body for delivery (ie, parturition) and lactation (ie, synthesis and secretion of milk). This lesson provides an overview of gestation, fetal circulation, parturition, and lactation. 10.4.01 Pregnancy As discussed in Lesson 10.2, the first stage of gestation (the **embryonic period**) occurs within the first eight weeks following fertilization. The embryonic period begins with zygote formation (week two of pregnancy), and by the end of this period, the embryo has initiated the development of all major organ systems. The **fetal period** begins at the ninth week after fertilization (week 11 of pregnancy) and lasts until birth. Relatively few new structures are formed during the fetal period, but fetal growth is significant during this time. Gestation is generally divided into three equal parts known as **trimesters**. The first trimester (weeks 1--12) includes fertilization, implantation, the embryonic period, and the first stages of the fetal period. By the second trimester (weeks 13--26), the placenta is fully formed and functions to sustain the fetus while secreting pregnancy hormones (eg, human chorionic gonadotropin, estrogen, progesterone). The third trimester begins at week 27 and lasts until the end of pregnancy (weeks 38--40). A timeline of the development of select embryonic and fetal structures during gestation is shown in Figure 10.20. Chapter 10: Pregnancy, Development, and Aging 360 **Figure 10.20** Timeline of the development of select embryonic and fetal structures during gestation. 10.4.02 Fetal Circulation The fetus acquires oxygen (O2) and nutrients and eliminates carbon dioxide (CO2) and waste through the maternal circulatory system. Once the fetal circulatory system is functional, fetal-maternal materials are exchanged through the **placenta**. The placenta is attached to the fetus at the **umbilicus** (ie, navel) via the **umbilical cord**, which is derived from the embryonic yolk sac and allantois (see Concept 10.1.02). Typically, there is no mixing of maternal and fetal blood because the exchange of materials occurs via diffusion through capillary walls. Fetal red blood cells contain a specialized type of hemoglobin (**fetal hemoglobin** \[**hemoglobin F**\]), which has a greater O2 affinity than adult hemoglobin (**hemoglobin A**), thereby favoring O2 transfer from the maternal blood supply to fetal blood (see Concept 13.1.03). Embedded in the uterine wall, the placenta contains many small [blood vessels](javascript:void(0)) emerging from the umbilical cord and branching into capillaries in the **chorionic villi** of the placenta (Figure 10.21). O2 and nutrients are transported from a uterine artery into the **intervillous space** (ie, space around the chorionic villi), and eventually diffuse into fetal capillaries connected to the unpaired **umbilical vein**. CO2 and wastes from fetal blood are transported via paired **umbilical arteries** into fetal capillaries at the placenta and diffuse into the intervillous space and subsequently into a uterine vein.  Chapter 10: Pregnancy, Development, and Aging 361 **Figure 10.21** Fetal and maternal circulation at the placenta. The placenta also functions as a protective barrier. Although most bacteria and viruses cannot cross the placenta, certain pathogens (eg, HIV, *Treponema pallidum* \[causes syphilis\], rubella virus \[causes German measles\]) can travel through the placenta, potentially leading to birth defects and/or fetal disease. Certain maternal [immunoglobulins](javascript:void(0)) (ie, antibodies) can cross the placenta, conferring partial pathogen immunity to the fetus, and are thought to function in fetal immune system \"training.\" Oxygenation of fetal blood occurs in the placenta, not the fetal lungs. Therefore, fetal circulation to and from the placenta is reminiscent of the [pulmonary circulation](javascript:void(0)), in which pulmonary veins carry oxygenated blood from the lungs to the heart and pulmonary arteries carry deoxygenated blood from the systemic circulation to the lungs (see Concept 13.1.07). After acquiring O2 and nutrients from the maternal circulation at the placenta, oxygenated fetal blood returns to the fetus via a single umbilical vein in the [umbilical cord](javascript:void(0)), and deoxygenated blood is transported into the placenta from the fetus via two umbilical arteries in the umbilical cord (Figure 10.22). Because some fetal organs (eg, lungs, liver) are not functional during gestation, there are several key differences between the fetal and postnatal circulatory systems. Oxygenated blood (ie, high O2 saturation \[SpO2\]) enters the fetus via the umbilical vein, which divides into two branches at the fetal liver. Most of the blood flows into one branch, the **ductus venosus**, which bypasses the liver and connects with the inferior vena cava. Deoxygenated blood (low SpO2) returning from the fetal systemic circuit enters the venae cavae, mixes with the oxygenated blood from the ductus venosus, and passes into the right atrium (Figure 10.22). A diagram of a human body AI-generated content may be incorrect. Chapter 10: Pregnancy, Development, and Aging 362 **Figure 10.22** Fetal circulatory system. An opening in the fetal heart called the **foramen ovale** acts as a one-way valve that connects the right and left atria. Most of the oxygenated blood entering the right atrium (ie, from the ductus venosus) passes through the foramen ovale into the left atrium and joins the systemic circulation via the aorta. The small amount of blood that passes into the right ventricle (rather than moving via the foramen ovale) is pumped into the pulmonary trunk and directly into the **ductus arteriosus**, a vessel that bypasses the lungs, connecting the pulmonary trunk directly to the descending aorta. This deoxygenated blood mixes with the O2-rich blood in the descending aorta, sending moderately oxygenated blood to the lower body of the fetus. Deoxygenated blood returning from the fetal systemic circulation is transported back to the placenta via the umbilical arteries (Figure 10.22). A summary of fetal circulatory structures and their functions can be found in Table 10.3.  Chapter 10: Pregnancy, Development, and Aging 363 **Table 10.3** Fetal circulatory structures. Directly following birth, the infant takes its first breaths, and O2 in the now-functional lungs signals the cessation of placental blood flow. In addition, breathing via the lungs leads to pressure changes that cause the ductus arteriosus, ductus venosus, and foramen ovale to close, a process that typically begins 12-24 hours after birth. 10.4.03 Parturition Typically, when a pregnancy has reached full term (38--40 weeks), the fetus exits the uterus through the vagina, a process known as **parturition** (ie, childbirth). **Labor** is the process by which childbirth occurs and is triggered by hormones from both the placenta and the fetus, along with mechanical cues. The initiation of labor is not well understood, but the process likely depends on a combination of maternal and fetal signals. When labor begins, increased pressure on the cervix causes impulses to be sent to neurosecretory glands in the hypothalamus, causing the hormone [oxytocin](javascript:void(0)) to be secreted via the posterior [pituitary](javascript:void(0)) [gland](javascript:void(0)). Oxytocin secretion stimulates the release of **prostaglandins** in the uterine myometrium, causing uterine contractions. A **positive feedback** loop is thus initiated: Each contraction causes more pressure on the cervix, sending increased signals for oxytocin release. When the infant is born, the positive feedback loop is broken because pressure on the cervix is relieved, as illustrated in Figure 10.23. A white sheet with black text AI-generated content may be incorrect. Chapter 10: Pregnancy, Development, and Aging 364 **Figure 10.23** Hormonal control of parturition. Labor can be divided into three stages, as shown in Figure 10.24: 1.**Dilation of the cervix:** From the onset of regular uterine contractions, cervical dilation typically lasts 6--12 hours. During this time, the amniotic sac (ie, fluid-filled sac surrounding the fetus) is usually ruptured. Dilation of the cervix is considered complete at 10 cm. 2.**Expulsion:** Delivery of the infant through the vagina typically occurs within 10 minutes to several hours after complete cervical dilation. 3.**Delivery of the placenta:** The placenta separates from the uterine wall and is typically delivered via the birth canal within minutes to an hour after birth of the infant.  Chapter 10: Pregnancy, Development, and Aging 365 **Figure 10.24** Stages of labor. 10.4.04 Lactation Following childbirth, an infant can no longer rely on the placenta for nutrition and must depend instead on external sources, including breast milk. During puberty, the breasts develop under the influence of estrogen, but do not **lactate** (ie, produce milk). Near the end of pregnancy, the milk-producing **mammary glands** of the breasts develop further and are converted into secretory structures. Fully developed mammary glands are composed of 15--20 lobes, which secrete milk when stimulated. Before delivery, when estrogen and progesterone are high, the mammary glands produce small amounts of a thin, low-fat secretion called colostrum. Within days of delivery, when estrogen and progesterone are decreased, the mammary glands produce milk with a higher fat and calcium concentration than colostrum. Mammary glands also secrete immunoglobulins (ie, antibodies), providing passive immunity to the infant. When the infant latches to the breast, stimulation of mechanoreceptors in the nipples sends signals to the hypothalamus, which stimulates the anterior pituitary gland to secrete **prolactin** and the posterior pituitary gland to secrete **oxytocin**. Prolactin stimulates milk production, whereas oxytocin stimulates the **let-down reflex**, a positive feedback loop resulting in the ejection of milk through milk ducts in the nipples. When the infant stops suckling, the stimulus for hormone release ends, and milk is no longer ejected (Figure 10.25). A diagram of a baby in a womb AI-generated content may be incorrect. Chapter 10: Pregnancy, Development, and Aging 366 **Figure 10.25** Hormonal control of milk secretion. **Concept Check 10.4** Rank the following structures of the fetal circulatory system based on their O2 saturation (SpO2) from highest to lowest saturation: Ductus arteriosus Umbilical arteries Umbilical vein [**Solution**](javascript:void(0))  **Cellular Regeneration and Senescence** Introduction Fetal development ends at birth, but growth and development continue throughout the lifespan of the organism. The ability of a fully developed organism to regenerate tissues varies throughout life, declining with age. In response to cellular injury or damage, an organism may initiate a program of **tissue regeneration** or repair. Alternately, older or damaged cells may enter a **senescent** (ie, growth-arrested) state, with senescent cells accumulating as the organism ages. This lesson discusses the regenerative capabilities of mammalian tissues, as well as the effects of aging and senescence at both the cell and organism levels. 10.5.01 Regeneration With the assistance of adult stem cells, many [tissues](javascript:void(0)) possess self-renewing and self-repairing capabilities. **Tissue regeneration** is a program of cell proliferation and growth that renews some structures and tissues throughout life (eg, [endometrial lining](javascript:void(0)), [red blood cells](javascript:void(0)), [epidermis](javascript:void(0))) or restores damaged tissues with the same functional tissue present before the injury. For example, when the epidermis is injured, a series of regenerative steps leads to the ultimate [replacement](javascript:void(0)) of the damaged area with functional (ie, identical) epidermal tissue. However, some tissues (eg, nerve cells) cannot be replaced. Likewise, when certain tissues (eg, [cardiac muscle](javascript:void(0))) are injured, the injured tissue is replaced with scar (ie, fibrotic) tissue instead of functional tissue during **repair**. Figure 10.26 illustrates the differences between tissue regeneration and repair. **Figure 10.26** Tissue regeneration and repair. In general, mammalian tissues have limited regenerative capabilities. For example, it is impossible for humans to regenerate an amputated limb, but healing near the area of amputation via thick scar tissue is possible. In addition to healing bone fractures and replacing lost blood, humans can also regenerate several other tissues in the body (eg, liver). Although tissue renewal and repair involve the [differentiation of stem cells](javascript:void(0)), regenerating tissues are formed from local stem cell populations in the context of a fully developed organism, rather than in the context of [embryonic development](javascript:void(0)). The potency of adult stem cells is much more restricted than the potency of embryonic stem cells. Mammalian tissues are limited in their regenerative potential due to differences between the embryonic and adult cellular environments. A diagram of a disease AI-generated content may be incorrect. Chapter 10: Pregnancy, Development, and Aging 368 10.5.02 Cell and Organism Aging **Senescence** is a cellular response that limits the proliferation of aged or damaged cells (Figure 10.27). Although senescence plays a physiological role in tissue homeostasis during normal development, it is also a stress response triggered by events associated with aging (eg, [telomere shortening](javascript:void(0)), genome instability, mitochondrial dysfunction). For example, cells are limited to a finite number of divisions due to the shortening of telomeres with each round of cell division and when telomere lengths are shortened past a critical point, a program of cellular senescence may be initiated. Cellular senescence involves a series of programmed events including [chromatin remodeling](javascript:void(0)), metabolic changes, and increased [autophagy](javascript:void(0)), culminating in stable growth arrest of the senescent cell. Senescent cells often secrete pro-inflammatory [cytokines](javascript:void(0)), leading to both short- and long-term consequences (Figure 10.27). Senescence has numerous physiological roles. For example, a cellular senescence program can be implemented during normal embryonic development, in response to cellular injury, or in transformed (ie, cancerous) cells to prevent potential detrimental effects. **Figure 10.27** Short- and long-term effects of cellular senescence.  Chapter 10: Pregnancy, Development, and Aging 369 The role of senescence in cancer development is paradoxical. Senescence can be considered a mechanism of [tumor](javascript:void(0)) suppression, *limiting* the development of cancerous cells by removing the cells from the [cell cycle](javascript:void(0)). However, accumulation of senescent cells may also play a role in *promoting* tumor progression through the secretion of pro-inflammatory cytokines, increasing cancer incidence with age. In addition, cancer cells may begin expressing the enzyme [telomerase](javascript:void(0)), which can replenish telomere ends. Telomerase expression allows cells to divide indefinitely, thereby preventing a protective senescent state. Senescent cells are sometimes eliminated (ie, via [apoptosis](javascript:void(0))), but, in many cases, senescent cells can accumulate over time, leading to decreased organism function. The accumulation of senescent cells is thought to be a contributing factor in organism aging, likely due to the secretion of pro-inflammatory factors by senescent cells (Figure 10.27). As more and more cells enter a senescent state, stem cell numbers decline, leading to the tissue deterioration associated with aging. **Concept Check 10.5** A cell that has entered a state of senescence due to telomere shortening is able to re-enter a non-senescent (ie, active) state. Propose a possible mechanism by which this could occur. [**Solution**](javascript:void(0))