Biochemistry Notes Unit 1 PDF
Document Details
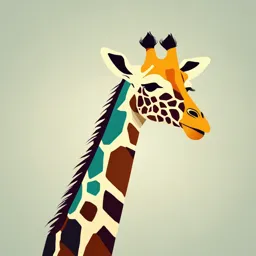
Uploaded by EncouragingLogarithm2639
University of Illinois Urbana-Champaign
Tags
Summary
These notes cover the fundamentals of biochemistry and includes topics such as the four classes of biomolecules, protein function and structure, nucleic acids, carbohydrates, and lipids. The content delves into various biochemical processes including how the different molecules works. Excellent resource for students.
Full Transcript
Unit 1 Biochem Notes Focus on what is blue and red in the lecture slides!!!! Lecture 1: Four Classes of Biomolecules: - Proteins - Nucleic Acids - Carbohydrates (poly and oligosaccharides) - Lipids (not a polymer) Proteins: - Linear polymers of L-amino aci...
Unit 1 Biochem Notes Focus on what is blue and red in the lecture slides!!!! Lecture 1: Four Classes of Biomolecules: - Proteins - Nucleic Acids - Carbohydrates (poly and oligosaccharides) - Lipids (not a polymer) Proteins: - Linear polymers of L-amino acids that are joined by peptide bonds - Amino acid is made from amino group, COOH, and a distinctive R chain for each amino acid - Alpha carbon is between the carboxyl and amino groups. - Peptide bonds are formed through condensation reactions - A condensation reaction is when water is removed between the COOH of one amino acid and NH2 of the next - Peptidyltransferase allows this condensation reaction to occur Protein Function: - Proteins are the most versatile biomolecules - They serve as signaling molecules; hormones, growth factors - They are transporters; iron, amino acid, glucose - They help with catalysis through enzymes - They aid in movement through actin myosin and tubulin - They aid in structure through collagen of tendons and cartilage, elastin of ligaments, keratin of hair, feathers, and nails, fibroin of silk and webs - Aid in gene regulation through transcription factors Nucleic Acids: - The information molecules of the cell - DNA is composed of deoxyribonucleotides - RNA composed of ribonucleotides - Nucleic acids are polymers of nucleotides that are joined by phosphodiester bonds - Phosphodiester bond: Covalent bond between nucleotides in DNA and RNA catalyzed by DNA or RNA polymerase - Condensation reaction: water eliminated between OH of nucleotide & phosphate of the next - Central dogma of molecular biology: information flows from DNA to RNA and then to protein. Carbohydrates: - Linear or branched polymers of monosaccharides (sugars) - Most sugars are in the cyclic form (ring form), not in the open chain (acrylic) form - Ribose are glucose are common sugars - If the aldehyde and OH are in the different direction, then alpha, if they are in the same direction then beta - Sugars are monosaccharides -There are thousands of different carbohydrates, which can be linked together in chains and form branches via glycosidic bonds (catalyzed by lactose or sucrose synthase) Carbohydrates functions: - Important fuel source (especially glucose, which is stored as glycogen in animals in the liver and muscle, and starch in plants) - Structural molecules (cellulose, chitin) - Signaling molecules: cell-cell recognition— carbohydrates are attached to proteins and lipids on the cell membranes, forming glycoproteins and glycolipids. (Immune cells, pathogens, blood group antigens) - Lubricants: hyaluronic acid in joints; mucus Lipids: - Water insoluble molecules that are highly soluble in organic solvents - Free fatty acids (nonesterified fatty acids): is most commonly used as a fuel - Triacylglycerols: The storage form of the fatty acids - Phospholipids: membrane lipids - Glycolipids: Bound to carbohydrates, membrane components - Steroids: they are polycyclic hydrocarbons, signaling molecule and membrane components Fatty Acids: - Main source of fuel - The simplest form of a lipid; long hydrocarbon chain and carboxyl group - Saturated fatty acid (single bond, found in meat, solid, long hydrocarbon chain) - Unsaturated fatty acid (double bond, common in natural fats, liquid) Triacylglycerols: - Storage form of fatty acids - Fats: consists of fats consisting of glycerol and 3 fatty acids. Fats are created via 3 condensation reactions creating ester linkages that link the fatty acid carboxyl groups to the hydroxyl groups in glycerol. - Ester bond (ester linkage) is present, also called esterification Membrane lipids: - Phospholipids: part of the molecule is hydrophilic, meaning it can dissolve in water, whereas the other part, made up of one or more hydrocarbon chains, is hydrophobic and cannot dissolve in water. Called an amphipathic molecule Covalent Bonds: - Bonds in which the electrons are shared by the participating atoms - Peptide bond: each amino acid is attached to another amino acid by a covalent bond—peptidyltransferase - Phosphodiester bond: covalent bond in RNA or DNA that holds a polynucleotide chain together - Ester bonds: Triglycerides are lipids consisting of one glycerol with three fatty acid molecules; bonded by ester bonds. - Glycosidic bond: Links monosaccharides together to make polysaccharide carbohydrates Noncovalent bonds: - Hydrogen bonds, ionic bonds, van der waals interactions, hydrophobic bonds Water: - Water is a dipolar molecules with an uneven distribution of electrons between the hydrogen and oxygen - Oxygen nucleus attracts e- more strongly than hydrogen, e- sharen unequally, e- more often in vicinity of oxygen. Unequal electron sharing results in dipoles - Forms hydrogen bonds with other polar molecules. - Universal solvent; very cohesive - Most common hydrogen bonds are OH and NH - Hydrogen bonds between two molecules will be disrupted by water, in as much as water itself forms hydrogen bonds with the molecules - The polarity of water and its ability to form hydrogen bonds renders it a solvent for any charged or polar molecule Electrostatic interaction: - A weak interaction between ions having opposite charges. Also called ionic bond or salt bridge. NaCl. Van Der Waals: - Very weak interaction between molecules that are neither polar nor charged - Short range attractive interactions - Depend on transient asymmetry in electrical charge induced by closely approaching atoms - Example: The binding of many enzymes with their specific substrates, the binding of each type of antibody with its specific antigen Hydrophobic interactions: - Aggregation of non-polar molecules results in an increase in randomness of water molecules - Hydrophobic effect: The process in which nonpolar molecules in aqueous solutions are driven together because of the resulting increase in entropy of water molecules - Second law of thermodynamics: The total entropy of the universe (system + surroundings) must increase in every spontaneous process - Membrane formation is powered by hydrophobic effect: the lipids form a bilayer with hydrophilic outside and hydrophobic interior - Protein folding is powered by hydrophobic effect: entails the transition from a disordered mixture of unfolded molecules to a comparatively uniform solution of folded protein molecules (nonpolar amino acids) Ionization of Water Molecules: - Water has a small but finite tendency to ionize and give H+ and OH- ions. Buffers: - Buffers are aqueous solutions that resist changes in pH as acid or base are added - Mixture of a weak acid (proton donor) and its conjugate base (proton acceptor) or a weak base and its conjugate acid - Buffer capacity is generally best within ~1 pH unit of pKa - Buffering maintains cellular pH and pH of body fluids Important Weak Acids: - Ammonia buffer: pKa= 9.25 - Phosphate buffer: pKa= 6.86 - Acetate buffer: pKa= 4.76 Lecture 2: Structural Features of Amino Acids: - Protein folding, binding to ligands, interaction with environment are all determined by side chain - Free amino acids have an alpha carbon that is between the carboxyl and amino groups - Side chain is distinctive for each amino acid and determines the properties of proteins - Peptidyl transferase: enzyme response for peptide bond formation Peptide Bonds: - Partial double bond character - Rigid and planar - Trans configuration - Uncharged but polar Types of Amino Acids: - Amino acids can be grouped into 4 groups on the basis of the chemical properties of the R groups 1. Hydrophobic Amino Acids: with nonpolar R groups 2. Polar Amino Acids: with neutral R groups but the charge is not evenly distributed 3. Positively charged Amino Acids: with R groups that have a positive charge at physiological pH (7.4) 4. Negatively charged amino acids: with R groups that have a negative charge at physiological pH Hydrophobic Amino Acids: - Nonpolar: have an even distribution of electrons, does not gain or lose proteins or participate in hydrogen or ionic bonds - Remain inside protein molecules - alanine (Ala), valine (Val), leucine (Leu), isoleucine (Ile), methionine (Met), phenylalanine (Phe), tryptophan (Trp), proline (Pro), and glycine (Gly) - Have side chains with only hydrogen and carbon - Alanine and Glycine are ambivalent, meaning they can be inside or outside the protein molecule - Hydrophobic amino acids cluster together inside the protein Hydrophobic Amino Acids & Hydrocarbon Side Chains: - Side chain is bonded to both the alpha carbon and the nitrogen atom and limits the rotation, reduces structural flexibility of polypeptide regions - Found in bends in the protein chain, the formation of the fibrous structure of the collagen. Location of Amino Acids in Proteins: - Nonpolar amino acids cluster in the interior of soluble proteins (soluble protein) - Nonpolar amino acids cluster also on the surface of membrane proteins (membrane protein) - Polar amino acids cluster on the surface of soluble proteins (soluble protein) Polar Amino Acids: - Have side chains with an electronegative atom (oxygen) - Hydroxyl groups (-OH) - (-OH), NH2 participate in hydrogen bonds - Phosphate group can be added to OH group via phosphorylation - Sugar can be added to the amide in glycoproteins - At slightly basic pH, the thiol group (SH) cab be oxidized to form disulfide bond (S-S) - Glycine (Gly), serine (Ser), threonine (Thr), cysteine (Cys), tyrosine (Tyr), asparagine (Asn), and glutamine (Gln) Positively Charged Amino Acids: - Hydrophilic (nitrogen) - Lysine, Arginine, Histidine - Histidine is often found in enzyme sites Negatively Charged Amino Acids: - Have acidic side chains - Aspartate is the ionic form of aspartic acid, glutamate is the ionic form of glutamic acid - Second carboxylic acid group on side chain is deprotonated at neutral pH - Ability of Asp and Glu side chains to donate protons can be important in enzyme catalysis - Aspartate and Glutamate Sickle Cell Disease: - Causes cells to look like a C-shaped tool; abnormal hemoglobin and wrong cell shape - Caused by mutation of the beta globin gene that causes a substitution of a valentine for a glutamate residue at the sixth position of the beta globin chain of hemoglobin - Glutamate carries a negative charge on its side chain at physiologic pH and thus can engage in ionic bonds or hydrogen bonds with water or other side chains - Valine is a hydrophobic amino acid and therefore tends to interact with other hydrophobic side chains to exclude water Structures of Proteins: - Primary: amino acid residues, sequence of a chain of amino acids - Secondary: alpha helix, local folding of the polypeptide chain into helices or sheets - Tertiary: three-D folding pattern of a protein due to a side chain interaction, polypeptide chain - Quaternary: assembled subunits, protein consisting of more than one amino acid chain Secondary Structure: - Alpha helix, hydrogen bond - A common structural motif in proteins in which a polypeptide main chain forms the inner part of a right handed helix - The side chains extending outward - Helix is stabilized by intrachain hydrogen bonds between amino and carboxyl groups of main chain - Hydrogen bonds, between AAs not next to each other but 4 AA apart Amino Acids that Disrupt Alpha Helix in Secondary Structures: - Proline: helix breaker, often interrupts the alpha helices found in globular proteins. - Rotation N-Alpha Carbon not possible - Not hydrogen bond formation - Large numbers of charged amino acids: Serine, aspartate, and asparagine - Amino Acids with bulky side chains (tryptophan) or with branch side-chain (valine, isoleucine), aliphatic amino acids Secondary Structure Beta Sheets: - A common structural motif in which a number of beta strands (multiple) are associated as stacks of chains - Stabilized by interchain hydrogen bonds - Running in the same direction or opposite directions to form an antiparallel pleated sheet - The alpha helix and beta sheet structures provide maximal hydrogen bonding for peptide bond components within the interior of polypeptides Tertiary Structure of Globular Proteins: - Driving force: hydrophobic interaction or hydrophobic effect - Covalent and other noncovalent interaction between side chains also contribute to the 3-D structure - Hydrophobic interactions: clustering of hydrophobic groups AWAY from water - Van der waals interactions - Hydrogen bond between side chains along with ionic bond and disulfide bridge Quaternary Structure of Proteins: - Refers to the association of individual polypeptide chain subunits in a geometrically and stoichiometrically specific manner: nonpolar side chains, hydrogen bonds, ionic bonds - Human hemoglobin, the oxygen-carrying protein in in blood, consists of two subunits of one type (designated α) and two subunits of another type (designated β) Globular, Fibrous, and Membrane Proteins: - Globular: Soluble and compact. Many are cytoplasmic & some secreted from cells (hemoglobin and myoglobin) hydrophobic residues inside - Fibrous: water insoluble, fiber-like, long strands or sheet repeating unit of 2-D structure, many similar polypeptides, tightly packed strong and structural proteins (collagen, keratin, myosin), silk fibroin, many in the form of beta sheet - Membrane: Embedded in membranes, many have more than 1 alpha helix stretches that span a membrane, hydrophobic residues. Protein Folding: - Follows specific folding pathways - Denatured, 2D structure formation, molten globule, to native - ~90 nsec - Unfolded/denatured is primary structure, native is tertiary Protein Denaturation: - Transformation of a well-defined folded protein structure under physiological condition, to an unfolded state under un-physiological condition disrupts the weak forces responsible for 3-D structure, leading to loss of structure and protein function - Denaturation by heat affects weak interactions in a protein such as hydrogen bonds - Characteristic melting temperature of a protein (Tm) is the temperature at which the transition from folded to unfolded happens (50% folded, 50% unfolded) - Goes from soluble to insoluble in heat Denaturation by pH: - Acids and bases disrupt salt bridges held together by ionic charges/bonds - Most proteins are denatured by acid or base because of electrostatic repulsion between side chains that arises if no more neutralization by opposite charges. - Gastric Acids (HCl) Denaturation by Chemical Urea: - Urea: reversible denaturing agent - Forms H-bonds to NH and C=O in peptide bonds, blocks intramolecular H-bonding - Interferes with hydrophobic interactions that normally stabilize the protein - “Dissolves out” the peptide chain - Reversible: dialyze away Urea, get refolding - Disulfide bond is broken down by a reducing agent: beta-mercaptoethanol Protein Misfolding and Disease: Amyloidosis: - General term for diseases in which a protein becomes misfolded and insoluble due to… - A mutation in the protein itself (sickle cell) - Some defect in post-translational processing of the protein causing the formation of protein aggregates or tangles called amyloid fibrils or plaques which usually adopt a beta sheet structure - Causes an alpha helical to beta sheet transformation. - Makes a non functional insoluble protein Lecture 3: - When the monomeric units (building blocks) in proteins, carbohydrates and nucleic acids are joined, the elements of H2O are eliminated. Overview of Protein Digestion: 1. Protein digestion is begun in the stomach by HCl and enzyme pepsin 2. Protein digesting enzymes are secreted from the pancreas into the small intestine 3. The small intestine is the major site of protein digestion; a little amount of dietary protein is lost in the feces 4. Final digestion of dipeptides and tripeptides to amino acids occurs inside the intestine 5. Absorbed amino acids enter the blood and travel to the liver 6. The liver regulates the distribution of amino acids to the rest of the body Stomach: - Dietary protein - First place for protein digestion - HCl (secreted by parietal cells) - Pepsin (secreted by gastric chief cells) Small intestine: - Polypeptides and amino acids broken down - Pancreas enzymes (trypsin, chymotrypsin, elastase, carboxypeptidases) - Oligopeptides and amino acids broken down next - Brush border epithelial cells The role of HCl in Digestion: 1. Protein denaturation: gastric acids (HCl and parietal cells) - Acids and bases disrupt salt bridges held together by ionic charges/bonds. They also prevent hydrogen bonding 2. Stomach: Pepsinogen is activated by HCl and pepsin - HCl Prepares the Substrate: Acid denatures proteins, exposing peptide bonds and making them accessible to enzymatic cleavage. - Pepsin Does the Cutting: Pepsin then hydrolyzes the peptide bonds, breaking proteins into smaller fragments that can be further digested in the small intestine by enzymes like trypsin and chymotrypsin. Secretion and Activation of Proteases: 1. Stomach: Pepsin is activated by HCl - Dietary Protein → Pepsin → Polypeptides and amino acids 2. Small intestine: Pancreatic enzymes are activated by enteropeptidase and proteases 3. Activation of proteases: Proenzymes (zymogens) → Active enzymes - Enteropeptidase is a trigger enzyme: triggers the activation of other digestive enzymes. It's a serine protease that's produced in the duodenum and secreted into the small intestine. Summary: Protein digestion begins in the stomach, where pepsin, activated by hydrochloric acid (HCl), breaks down dietary proteins into polypeptides and amino acids. In the small intestine, digestion continues as pancreatic proenzymes (zymogens), such as trypsinogen and chymotrypsinogen, are activated by enteropeptidase and other proteases into their active forms, like trypsin and chymotrypsin. These enzymes further cleave polypeptides into smaller peptides and free amino acids, which can be absorbed by the intestinal lining for use in the body. The sequential activation and function of these enzymes ensure efficient protein breakdown and nutrient absorption. Transport Amino Acids into Cells: 1. From intestinal lumen into epithelial cells: - Na+ dependent transport of amino acid - Na+ is pumped out on the serosal in exchange for K+ by the Na+, K+, ATPase 1. From epithelial cells into blood: - The amino acid is carried by a facilitated transporter down to its concentration gradient into the blood Amino acid transporter systems: - Transporter system: neutral amino acid (deficit in Trp) causes Hartnup disease - Dibasic amino acids: Deficit in cystine: causes Cystinuria or dibasic aciduria Neutral Amino Aciduria: Hartnup Disease: - Deficiency of Tryptophan, an essential amino acid - Genetic deficit in the transporter genes, which is responsible for the absorption of neutral or nonpolar amino acids - Loss of amino acids in the body, particularly, Tryptophan, which can be converted to serotonin melatonin, and niacin Cystinuria: - The most common genetic error of amino acid transporter (dibasic amino acids) - Cystinuria is an aminoaciduria in which large amounts of cystine is found in urine - Found in kidney - Cysteine and cystine have very different solubility and this is important medically - The body’s inability to reabsorb cystine leads to accumulation and subsequent precipitation of stones of cystine in the urinary tract Digestion of Carbohydrates: - Sites of breakdown: 1. Mouth: Digestion of carbohydrates starts from the mouth by salivary alpha-amylase. - Breaks down alpha-1,4-glycosidic bond 2. Small intestine: alpha amylase (salivary or pancreatic): only break down alpha-1,4-glycosidic bonds. Summary of Carbohydrate Digestion: - Carbohydrate digestion begins in the mouth, where salivary alpha-amylase breaks down starch and glycogen by hydrolyzing alpha-1,4 glycosidic bonds, producing smaller polysaccharides and maltose. - This process is halted in the acidic environment of the stomach but resumes in the small intestine, where pancreatic alpha-amylase, secreted by the pancreas, continues breaking down alpha-1,4 glycosidic bonds. - The resulting disaccharides and oligosaccharides are further broken down by brush border enzymes, such as maltase, sucrase, and lactase, into monosaccharides like glucose, fructose, and galactose, which are absorbed into the bloodstream via intestinal epithelial cells. Lactose Intolerance: - Reduced levels of lactase due to silencing of lactase gene - 90% of adults of African or Asian descent - Osmotically active: 1. Lactose 2. 2-carbon metabolites 3. 3-carbon metabolites Digestion of Dietary Lipids: - Sites: stomach and small intestine - Enzymes: lingual lipase in mouth, gastric lipase in stomach - Pancreatic enzymes: lipase cholesterol esterase, phospholipase A2, lysophospholipase Lipid Digestion in Stomach: - Enzymes are produced at the back of the tongue or in the stomach - Acid stable and remove short lipid chains (lingual lipase) and medium chains (gastric lipase) aka longer than 12 carbon chains fatty acids from TAGs - Most active in infants and young children who drink cows’ milk - Emulsification: occurs in the stomach mechanical mixing, increasing the surface area of the hydroponic lipid droplets Emulsification of Lipid in Small Intestine: - Emulsification of dietary lipids occurs in the duodenum and it increases the surface area of the hydrophobic lipid droplets so the digestive enzymes can act effectively - Emulsification is accomplished by two complementary mechanisms 1. Physical: Mechanical mixing due to wave-like movement of the intestine 2. Chemical: use of the detergent properties of the bile salts (amphipathic) - Unconjugated bile salt: insoluble in water, non-polar (hydrophobic) - Conjugated bile salts (taurine and glycine) Emulsification of Lipid by Bile Salts: - The bile salts break up (emulsify) the fat globules into smaller hydrophilic coated droplets that more readily mix with water - In the small intestine, fats are broken down through a process called emulsification, where large fat droplets are broken into smaller ones to make digestion easier. This is done with the help of bile, a substance made by the liver and stored in the gallbladder, which contains bile salts that act like detergents to keep the fat droplets from clumping together. Once emulsified, enzymes like pancreatic lipase can break down the fats into smaller molecules, such as fatty acids and glycerol, which the body can absorb. End Products of Lipid Digestion: - Primary products in the lumen: 1. Free fatty acids (16-18 C) 2. 2-monoacylglycerol 3. Cholesterol Micelles and Lipid Absorption: - Micelles, tiny microdroplets that are emulsified by bile salts, solubilizing lipid - Micelles contain majority fatty acids and 2-monoacylglycerols. Other dietary lipids, such as cholesterol, lysophospholipids, and fat soluble vitamins - Disc shaped clusters of amphipathic lipids, arranged with their hydrophobic groups on the inside and their hydrophilic groups on the outside - The micelles travel through a layer of water to the microvilli on the surface of the intestinal epithelial cells where the fatty acids, 2-monoacylglycerols, and other dietary lipids are absorbed, but the bile salts are left behind in the lumen of the gut - Short and medium chain fatty acids (C4 to C12) do not require bile salts for their absorption. Assembly and Secretion of Chylomicrons: - In the small intestine, after fats are digested into fatty acids and monoglycerides, they are absorbed by intestinal cells and reassembled into triglycerides. These triglycerides are combined with cholesterol, proteins, and other lipids to form chylomicrons, which are tiny particles that transport fats. The chylomicrons are packaged inside the cells and then released into the lymphatic system through tiny vessels called lacteals. From there, they eventually enter the bloodstream, delivering fats to tissues for energy or storage. - Assembly of chylomicrons inside the golgi apparatus - Newly synthesized TG and cholesterol ester as packaged as lipid droplets surrounded by a thin layer of Maturation of Chylomicrons: - When chylomicrons (fat carriers) are first made in the intestine, they are not fully ready to work and are called nascent chylomicrons. They have a key protein called ApoB that helps hold them together. To fully mature, they need two more proteins: ApoCII, which activates an enzyme (lipoprotein lipase) to break down fats inside the chylomicron, and ApoE, which helps the liver recognize and remove used chylomicrons from the blood. - The particle released by the intestinal mucosal cell (nascent chylomicron) is functionally incomplete. ApoB is the primary organizing protein - ApoCII is needed for the activation of lipoprotein lipase, the enzyme that degrades triglyceride contained in chylomicron - ApoE is recognized by hepatic receptors - High density lipids good, low density bad, because they cannot transport the chylomicrons Lecture 4: Degradation of Amino Acids: - Removal of the alpha-amino group (nitrogen) by transamination and oxidative deamination - OR Carbon skeletons of the alpha-keto acids are converted to common intermediates Nitrogen Removal is First Step: - Transamination: re-use for biosynthesis AA, nucleotides and biological amides - Transamination is the transfer of the alpha amino group of an AA to an alpha keto acid - End products are alpha keto acid and amino acid - Enzyme: Aminotransferases: cytosolic proteins in liver, kidney, intestine, and muscle - Coenzyme: Pyridoxal phosphate (PLP derivative of vitamin B6) is the cofactor. PLP is also a co-factor for reactions in deamination, decarboxylation, and gluconeogenesis. Summary: - Breaking down amino acids starts with removing the nitrogen part through a process called transamination. This means the nitrogen is moved from one molecule to another, helping the body make new proteins and other important substances. An enzyme called aminotransferase, found in different organs like the liver and muscles, helps with this process. It also needs a helper molecule called PLP, which comes from vitamin B6, to work properly. PLP is also useful in other body processes like breaking down molecules and making energy. Tissue Damage & Transamination: - When tissues are damaged, enzymes from inside cells leak into the blood. ALT is mainly found in the liver and is a key sign of liver damage. AST is found in the liver, heart, muscles, kidneys, brain, and red blood cells, so its presence in the blood can indicate damage to these organs. - This relates to transamination because ALT (alanine aminotransferase) and AST (aspartate aminotransferase) are aminotransferase enzymes, which play a key role in transamination—the process of transferring amino groups between amino acids and keto acids. - ALT catalyzes the transfer of an amino group from alanine to alpha-ketoglutarate, forming pyruvate and glutamate. - AST catalyzes a similar reaction with aspartate, forming oxaloacetate and glutamate. - When liver or muscle cells are damaged, these enzymes leak into the blood, making them useful markers for diagnosing organ damage. Removal of Nitrogen via Oxidative Deamination: - Place: liver and kidney - Enzyme: glutamate dehydrogenase - Oxidative deamination is a process where the amino group (-NH₂) is removed from an amino acid, releasing ammonia (NH₃) and converting the remaining part into a keto acid. This mainly happens in the liver and helps the body get rid of excess nitrogen. The enzyme glutamate dehydrogenase plays a key role by acting on glutamate, turning it into alpha-ketoglutarate while producing ammonia and NADH (energy molecule). The ammonia is then processed into urea and excreted. This step is important because it helps control nitrogen levels in the body. Urea Cycle: - Ammonium ion is converted into urea 1. The major disposal form of amino groups derived from amino acids 2. 90% of the nitrogen-containing components of urine 3. Place of production: liver 4. Place of excretion: kidney - The urea cycle is the body's way of getting rid of excess nitrogen from amino acid breakdown. It happens in the liver and converts toxic ammonia (NH₃) into urea, which is safely removed through urine. The cycle starts when ammonia and carbon dioxide combine to form carbamoyl phosphate, which then enters a series of steps involving molecules like ornithine, citrulline, and arginine. These reactions help attach nitrogen to urea, which is then sent to the kidneys for excretion. This process is crucial because it prevents ammonia from building up in the body, which can be harmful. Urea Cycle & Mitochondria and Cytosol: - The urea cycle takes place in both the mitochondria and the cytosol of liver cells. The first two steps happen in the mitochondria, where ammonia (NH₃) and carbon dioxide (CO₂) combine to form carbamoyl phosphate. - Then, carbamoyl phosphate joins ornithine to make citrulline, which moves out into the cytosol. - In the cytosol, citrulline goes through more reactions, adding another nitrogen from aspartate and forming arginine. - Finally, arginine is broken down to release urea (which is excreted) and ornithine, which returns to the mitochondria to restart the cycle. This movement between mitochondria and cytosol is essential for the process to function properly. - 4-high energy phosphates are consumed, making synthesis of urea an irreversible process BUN Test: - Blood Urea Nitrogen (BUN) test - 7-21 mg per deciliter is normal - High mg = kidney damage - Low mg= liver damage Nitrogen Metabolism in Hibernating Bears: - Hibernating bears do not eat, drink, urinate, or defecate, but they still use fat stores for energy, with ketone bodies fueling the brain. Despite not eating, their bodies continue protein turnover, recycling nitrogen efficiently. Urea is first sent to the bladder but is then reabsorbed into the blood and released into the intestine, where bacteria break it down into ammonium (NH₄⁺). This ammonium is used to make new amino acids and proteins, helping the bear maintain muscle and tissue. Additionally, breaking down urea creates energy by forming an electrochemical gradient used to make ATP. Amino Acid Carbon Skeletons: - When amino acids are broken down, their carbon skeletons can follow two main paths. Some amino acids are glucogenic, meaning they turn into pyruvate or TCA cycle intermediates, which can be used to make glucose for energy. - Others are ketogenic, meaning they break down into acetoacetate, acetyl-CoA, or acetoacetyl-CoA, which are used to produce ketone bodies like acetoacetate, 3-hydroxybutyrate, and acetone. - These ketone bodies provide an alternative energy source, especially when glucose is low. The TCA cycle plays a key role in processing these carbon skeletons for energy production. Aminotransferase: - Alanine is converted to pyruvate - Serine is converted to pyruvate - Serine comes from the glycine - Aspartate can be converted to oxaloacetate - Histidine can form alpha-ketoglutarate - Phenylalanine is hydrolyzed by phenylalanine hydroxylase to form tyrosine Histidine Degradation: - Histidine is broken down in the body through a process involving the enzyme histidase, producing FIGLU (N-Formiminoglutamate) as an intermediate. FIGLU is then converted into 5-formimino-tetrahydrofolate (THF), which requires folic acid (Vitamin B9). If Vitamin B12 or folic acid is deficient, FIGLU builds up and is excreted in the urine, which is why the FIGLU test is used to detect these deficiencies or possible liver disease. Phenylalanine to Tyrosine Illnesses: - Phenylketonuria (PKU) is a genetic disorder where the body cannot properly convert the amino acid phenylalanine into tyrosine due to a defect in the enzyme responsible for this conversion. This leads to a buildup of phenylalanine in the blood (hyperphenylalaninemia) and a shortage of tyrosine. Tyrosine is important for producing catecholamines, tissue proteins, and melanin (which affects skin pigmentation). The condition can also impact the production of BH4 (tetrahydrobiopterin), a cofactor involved in these reactions. If untreated, PKU can lead to intellectual disabilities and albinism (lack of pigmentation), due to a shortage of tyrosinase, the enzyme involved in melanin production. - Defect in conversion of AA can also cause “mousy” odor in urine Amino Acid Degradation: - Some amino acids, like valine, isoleucine, leucine, methionine, and threonine, are broken down into a molecule called succinyl CoA, which is used in the energy-making process in cells. For branched-chain amino acids (valine, isoleucine, leucine), the breakdown happens in two steps: first, the amino acid gets converted into a similar molecule by an enzyme called branched-chain aminotransferase, and then it gets further broken down by another enzyme called branched-chain α-keto acid dehydrogenase. - If the second enzyme doesn't work properly, it causes a condition called Maple Syrup Urine Disease (MSUD). For methionine, it’s first changed into homocysteine and plays an important role in transferring methyl groups (which are important for many reactions) through a molecule called S-adenosylmethionine (SAM). SAM: - S-adenosylmethionine (SAM) is an important molecule that donates methyl groups in many biochemical reactions, a process called one-carbon metabolism. These methyl groups are used in over 40 different reactions in the body to modify other molecules. The first step in making SAM is converting an amino acid called methionine into another molecule called homocysteine. This process is crucial because it helps in regulating gene expression, building DNA, and producing important molecules. Two Major Disposal Pathways for Homocysteine: - Synthesis of Cysteine & Resynthesis of Methionine - Homocysteine is an intermediate compound in the metabolism of the amino acid methionine. Its role is crucial for the body to recycle and maintain proper levels of methionine, which is important for various biological processes, especially those involving methylation (adding a methyl group to molecules like DNA, proteins, and lipids). Methylation affects gene expression, protein function, and other cellular processes. - However, high levels of homocysteine can be harmful, as it has been linked to an increased risk of cardiovascular diseases, nerve damage, and other health problems. This is why it's important for the body to regulate its levels properly, either by converting it to cysteine (for protein synthesis) or recycling it back to methionine. Two Disposal Pathways: 1. Synthesis of Cysteine: Homocysteine can be converted into cysteine, an amino acid. This process involves adding a sulfur group, and it helps in the production of proteins and other important molecules in the body. 2. Resynthesis of Methionine: Homocysteine can also be converted back into methionine. This process requires the addition of a methyl group, which is usually donated by a molecule called methyl-tetrahydrofolate (methyl-THF). This recycling of methionine is crucial to maintaining normal cellular function and methylation processes. Vitamin B12 is involved in two important reactions: 1. Resynthesis of Methionine from Homocysteine: Vitamin B12 (in the form of methylcobalamin) helps convert homocysteine back into methionine. This process requires tetrahydrofolate (THF) to donate a methyl group. The enzyme homocysteine methyltransferase facilitates this conversion. This reaction is important because methionine is essential for various cellular processes, including protein synthesis and methylation. 2. Synthesis of Succinyl CoA: Vitamin B12 (as deoxyadenosylcobalamin) is also involved in converting methylmalonyl CoA into succinyl CoA through the enzyme methylmalonyl CoA mutase. This reaction is crucial for energy production and the TCA cycle. If vitamin B12 is deficient, methylmalonyl CoA accumulates, and methylmalonic acid levels rise, which is used as a clinical marker for B12 deficiency. CoA: - CoA is a foundational molecule that helps transfer energy-rich groups like acetyl groups, Acetyl-CoA is an important energy molecule used in metabolic cycles, and Acetoacetyl-CoA is a building block for producing ketone bodies during times of energy stress. FlGlu test: - The FIGLU test (formiminoglutamate test) is used to diagnose folic acid (vitamin B9) deficiency because folate plays a key role in the metabolism of histidine, an amino acid. - When there's enough folate, histidine is broken down normally through a process that involves converting N-formiminoglutamate (FIGLU) into other substances. However, in a folic acid deficiency, this conversion is impaired. As a result, FIGLU accumulates in the body and is released into the urine. - The FIGLU test measures the amount of FIGLU in the urine. High levels of FIGLU indicate a deficiency of folic acid, since without enough folate, the body cannot properly process histidine, leading to the accumulation of FIGLU. Thus, this test is useful for detecting folic acid deficiency. Ammonia Fixation vs Nitrogen Fixation: - Ammonia Fixation: This refers to the process of incorporating ammonia (NH₃) into organic molecules, such as amino acids. In the body, this happens when ammonia is combined with other molecules (like α-ketoglutarate) to form amino acids like glutamate. - Nitrogen Fixation: This is a biological process in which atmospheric nitrogen (N₂) is converted into a more usable form, like ammonia (NH₃), by certain bacteria (like those in the soil or in the roots of leguminous plants). This process is crucial for providing nitrogen to plants, which is then incorporated into proteins and other molecules. Nitrogen fixation is performed by nitrogen-fixing bacteria and is a vital part of the nitrogen cycle. - In short, ammonia fixation deals with incorporating ammonia into organic molecules, while nitrogen fixation involves converting atmospheric nitrogen into ammonia. - Ammonia fixation is about using ammonia inside the body, and nitrogen fixation is about turning nitrogen from the air into a usable form for plants. Keto acids for the formation of alanine, aspartate and glutamate: - Alanine: The corresponding keto acid is pyruvate. Alanine is formed from pyruvate through a transamination reaction. - Aspartate: The corresponding keto acid is oxaloacetate. Aspartate is formed from oxaloacetate via transamination. - Glutamate: The corresponding keto acid is α-ketoglutarate. Glutamate is formed from α-ketoglutarate through transamination. Lecture 5: Nucleic Acids: - The information molecules in the cell Nucleotide: - Nucleotide is composed of phosphate group(s), a nitrogenous base (purine or pyrimidine), and a pentose sugar Purines: Adenine and Guanine Pyrimidines: Cytosine and Thymine (and Uracil) Nucleoside: - Nitrogen base + sugar De Novo and Salvage Pathways: - In De Novo synthesis, the base itself is synthesized from simple starting materials, including amino acids - In Salvage pathways, preformed bases are recovered and attached to an activated ribose - Summary: Cells make nucleotides in two ways: De Novo synthesis builds the nucleotide bases from scratch using small molecules like amino acids, while the Salvage pathway recycles already-made bases by attaching them to a sugar-phosphate (activated ribose). The De Novo pathway is useful when cells need to create nucleotides from basic building blocks, whereas the Salvage pathway saves energy by reusing existing bases from broken-down DNA and RNA. Synthesis of Purine Nucleotides: - The purine ring is constructed by a series of reactions that add carbons and nitrogen to preformed ribose 5-phosphate - Purine nucleotides are built step by step on a ribose 5-phosphate backbone. The process starts with the formation of PRPP (5-phosphoribosyl-1-pyrophosphate), an activated form of ribose, using PRPP synthetase, which requires energy stored in pyrophosphate. - The purine ring is then assembled by adding carbon and nitrogen atoms from various sources, including ATP (energy), N10-formyl-THF (one-carbon donor), and amino acids. - This leads to the formation of inosine monophosphate (IMP), the first purine nucleotide, which can later be converted into AMP (adenosine monophosphate) or GMP (guanosine monophosphate). The enzyme ribonucleotide reductase then converts ribonucleotides and deoxyribonucleotides for DNA synthesis. Regulation of Purine Synthesis: - Purine synthesis is carefully controlled to prevent wasteful overproduction. The enzyme PRPP synthetase, which helps start the process, is inhibited by purine nucleotides (AMP, GMP, and IMP) through negative feedback regulation—meaning when enough purines are available, they signal the enzyme to slow down. This ensures the cell only makes purines when needed, balancing energy use and nucleotide supply. XMP (xanthosine monophosphate) also plays a role in regulating purine levels by acting as an intermediate in GMP production. Azathioprine Drug: - Azathioprine is a purine analogue used as an immunosuppressive and cancer drug because it interferes with purine synthesis, preventing rapidly dividing cells (like immune cells or cancer cells) from making DNA. Other synthetic inhibitors of purine synthesis target key enzymes in the pathway. For example, PRPP synthetase is regulated by negative feedback from purine nucleotides (AMP, GMP, IMP) and is also inhibited by mycophenolic acid, a drug that suppresses the immune response by blocking GMP production. These regulatory mechanisms and inhibitors help control purine levels in the body. Salvage Pathway for Purines: - Turnover of cellular nucleic acids and nucleic acids from diet - In salvage pathways, preformed bases are recovered and attached to an activated ribose PRPP - In the salvage pathway for purines, the body recycles preformed purine bases from the breakdown of cellular nucleic acids or from the diet. These bases are recovered and then attached to an activated ribose molecule (PRPP), which helps form new purine nucleotides. This process conserves energy by reusing existing purine bases, rather than synthesizing them from scratch, and ensures a steady supply of nucleotides for cellular functions like DNA and RNA synthesis. Lesch-Nyhan Syndrome - Deficiency of HGPRT - Increased PRPP and decreased IMP and GMP, leading to an increase in De Novo synthesis - Increased breakdown of purine, therefore, increased uric acids - Excessive production of uric acid (hyperuricemia) Degradation of Purine Nucleotides: - AMP (adenosine monophosphate) and GMP (guanosine monophosphate) are purine nucleotides that play essential roles in cellular functions like DNA and RNA synthesis. These purines can be synthesized de novo or recycled through the salvage pathway, where preformed purine bases are attached to activated ribose (PRPP) to form nucleotides. Uric acid is the end product of purine metabolism, formed when purines are broken down. - Excess purine breakdown can lead to high levels of uric acid, which may cause gout if it crystallizes in joints. - Thus, the regulation of purine nucleotide synthesis and degradation is critical for maintaining balance in cellular processes. Gout: - High levels of uric acid in the blood due to underexcretion or overproduction of uric acid - Deposition of crystals of sodium urate in kidney and joint, causing pain and inflammation Gout Treatment: - Prevent the deposition of urate crystals - Colchicine: Decrease the movement of the granulocytes into the deposition area (inhibition of inflammation) - Inhibition of uric acid production: Allopurinol Sources of Purine Nucleotides: - Dietary purine - Tissue nucleic acids - Endogenous purine synthesis Adenosine deaminase deficiency (ADA): - Severe combined immunodeficiency (SCID) - This enzyme regulates the breakdown of purines & when it doesn't work there are issues - ADA deficiency (Adenosine Deaminase deficiency) is a genetic disorder that impacts the immune system, leading to Severe Combined Immunodeficiency (SCID). - ADA is an enzyme involved in purine metabolism, specifically in the conversion of deoxyadenosine to deoxyinosine. When ADA is deficient, deoxyadenosine accumulates and is toxic to developing T and B cells, which are crucial for immune function. - This accumulation also affects ribonucleotide reductase, an enzyme responsible for producing deoxyribonucleotides for DNA synthesis. - As a result, DNA synthesis is impaired, especially in immune cells, leading to SCID. - Treatment includes bone marrow, stem cell transplants, injection of ADA, and gene therapy. - Shortened life expectancy De Novo Pyrimidine Synthesis: - De novo pyrimidine synthesis begins with the construction of the pyrimidine ring using bicarbonate (CO₂), aspartic acid, and ammonia as sources of carbon and nitrogen. In this pathway, the pyrimidine base is synthesized first, and then it is attached to 5-phosphoribosyl-1-pyrophosphate (PRPP), an activated ribose molecule, to form a nucleotide. The first base synthesized in this process is orotate, which is later converted to uridine monophosphate (UMP). This process is essential for creating pyrimidine nucleotides (like UMP, CMP, and TMP), which are necessary for RNA and DNA synthesis. Salvage Pathways for Pyrimidine Synthesis: - Pyrimidine Salvage Pathway recycles preformed pyrimidine bases from the breakdown of nucleic acids to synthesize new nucleotides, saving energy compared to de novo synthesis. Thymine, a product of DNA degradation, is first converted into a nucleoside (thymidine) by thymidine phosphorylase. Then, thymidine kinase adds a phosphate group to thymidine, forming thymidine monophosphate (TMP), which can be further phosphorylated into TDP and TTP for DNA synthesis. This pathway ensures a steady supply of pyrimidine nucleotides needed for cell growth and repair. Chemotherapy Drug: - 5-fluorouracil Tetrahydrofolate: - Tetrahydrofolate (THF, FH₄) in Amino Acid Metabolism plays a crucial role in transferring one-carbon units for various biosynthetic reactions. 1. Degradation of Histidine – THF is involved in breaking down histidine, where it accepts a methylene (-CH₂-) group to form N⁵-formimino-THF, which later contributes to folate metabolism. 2. Serine Synthesis – THF helps in converting serine to glycine by donating or accepting one-carbon units, an essential reaction in amino acid and nucleotide metabolism. 3. Resynthesis of Methionine from Homocysteine – The enzyme homocysteine methyltransferase (methionine synthase) uses N⁵-methyl-THF as a methyl donor to convert L-homocysteine into methionine, with the help of methylcobalamin (Methyl-B12). This process is vital for maintaining methionine levels and preventing homocysteine buildup, which is linked to cardiovascular disease. 4. Overall, THF is essential for amino acid metabolism, nucleotide synthesis, and methylation reactions, supporting DNA synthesis and overall cellular function. One Carbon Pool: - The primary one-carbon carrier in the body is tetrahydrofolate (THF), which helps transfer and carry one-carbon units in different oxidation states (methane, methanol, formaldehyde, and formic acid). These transfers are essential for amino acid metabolism, nucleotide synthesis, and methylation reactions, supporting DNA production and overall cell function. Structure and Forms of Tetrahydrofolate: - The nutrient comes directly from food sources it is called folate (vitamin B9) - When it is manufactured for use as a supplement or to fortify foods, it is called folic acids Sulfonamides and Their Specificity: Sulfonamides are antibiotics that specifically target bacteria by inhibiting folate synthesis, which is essential for bacterial growth and replication. They work by blocking dihydropteroate synthase, an enzyme needed for producing tetrahydrofolate (THF). Since humans obtain folate from their diet rather than synthesizing it, sulfonamides selectively harm bacteria without affecting human cells. Roles of THF Derivatives in Metabolism: Histidine Metabolism (FIGLU Test) – THF is required to break down histidine into glutamate. If THF is deficient, FIGLU (formiminoglutamate) accumulates, which is used in diagnostic tests for folate deficiency. Serine Metabolism – THF helps convert serine to glycine, transferring a one-carbon unit for nucleotide synthesis. Methionine Metabolism – N⁵-methyl-THF donates a methyl group to homocysteine, converting it into methionine, a process vital for methylation reactions in the body. Major Carbon Source for One-Carbon Groups: The major carbon source for one-carbon units in humans is serine. It donates a methylene (-CH₂-) group to THF, forming N⁵,N¹⁰-methylene-THF, which is crucial for DNA synthesis and amino acid metabolism. Lecture 6: - There are four high energy phosphates that are consumed during the urea cycle; making the synthesis of urea an irreversible process Oxidative Phosphorylation: - Oxidative phosphorylation is the process by which cells generate ATP using energy from electrons transferred through the electron transport chain (ETC) in the mitochondria. - Electron Transfer – Electrons from NADH and FADH₂ are passed through protein complexes (Complexes I-IV) in the inner mitochondrial membrane. - Proton Pumping – As electrons move, protons (H⁺) are pumped into the intermembrane space, creating a proton gradient. - ATP Synthesis – Protons flow back into the matrix through ATP synthase (Complex V), driving the conversion of ADP to ATP. - Oxygen as Final Electron Acceptor – At the end of the chain, electrons combine with oxygen and protons to form water (H₂O). - This process efficiently produces ~30-32 ATP per glucose molecule, powering cellular functions. - The point of oxidative phosphorylation is to produce ATP, the main energy currency of the cell, by using energy from electron transfer. This process allows cells to efficiently convert glucose and fatty acids into a large amount of ATP (~30-32 ATP per glucose), which powers essential biological functions like muscle contraction, nerve signaling, and biosynthesis. Without oxidative phosphorylation, cells would rely on less efficient energy production methods, such as glycolysis, which generates only 2 ATP per glucose. Additionally, this process maintains a proton gradient that is crucial for mitochondrial function and overall cellular metabolism. Tricarboxylic Cycle: - The Tricarboxylic Acid (TCA) Cycle, also called the Krebs cycle or Citric Acid Cycle, is a key metabolic pathway that occurs in the mitochondrial matrix. It extracts energy from acetyl-CoA, which comes from carbohydrates, fats, and proteins, to generate ATP, NADH, and FADH₂ for cellular energy production. Mitochondria: - The mitochondrion is the powerhouse of the cell, with distinct compartments that carry out different metabolic processes: 1. Outer Membrane – Permeable to small ions and molecules due to mitochondrial porins, allowing passive diffusion. It is also involved in fatty acid (FA) elongation, desaturation, and phospholipid synthesis. 2. Inner Membrane – Highly folded into cristae to increase surface area, it is impermeable to most molecules. This is the site of electron transport, ATP synthesis, and fatty acid transport. Oxidative phosphorylation occurs here. 3. Intermembrane Space – Contains nucleotide kinases and maintains the proton gradient, which is crucial for ATP production. 4. Matrix – The innermost compartment where major metabolic pathways take place, including: Citric Acid Cycle (TCA) – Produces NADH and FADH₂ for energy production. - Fatty Acid Oxidation – Breaks down fats for energy. - Pyruvate Dehydrogenase – Converts pyruvate to Acetyl-CoA. - Urea Cycle – Detoxifies ammonia. - Mitochondrial DNA/RNA Transcription & Translation – Mitochondria have their own DNA and ribosomes for protein synthesis. - This organization allows mitochondria to efficiently generate ATP and regulate essential cellular processes. Electron Transport Chain: - The Electron Transport Chain (ETC) is a series of four protein complexes (I-IV) embedded in the inner mitochondrial membrane that transfer electrons from NADH and FADH₂ to oxygen, generating a proton gradient. This process is also called the respiratory chain and is essential for ATP production. Steps of the ETC: - Complex I (NADH-Q Oxidoreductase) – Accepts electrons from NADH, transfers them to ubiquinone (Q), and pumps protons (H⁺) into the intermembrane space. - Complex II (Succinate-Q Oxidoreductase) – Accepts electrons from FADH₂ but does not pump protons. - Complex III (Q-Cytochrome C Oxidoreductase) – Transfers electrons from Q to cytochrome C and pumps protons. - Complex IV (Cytochrome C Oxidase) – Transfers electrons from cytochrome C to oxygen, reducing it to water (H₂O), and pumps protons. Outcome of the ETC: - Reduction of oxygen (O₂) to H₂O. - Creation of a proton gradient in the intermembrane space, which drives ATP synthesis via ATP synthase in oxidative phosphorylation. - This process is critical for cellular energy production, allowing the synthesis of ATP, which powers various biological functions. ETC & Oxidation and Reduction: - The Electron Transport Chain (ETC) is a series of coupled oxidation-reduction reactions where electrons from NADH and FADH₂ are transferred through protein complexes in the inner mitochondrial membrane. As electrons move through the chain, their electron affinity increases, helping drive ATP synthesis. Key Electron Carriers in the ETC: - Flavin Mononucleotide (FMN) – Accepts electrons from NADH in Complex I. - Iron-Sulfur Proteins – Contain iron clusters that transfer electrons within Complex I, II, and III. - Coenzyme Q (Q, Ubiquinone) – A mobile electron carrier that shuttles electrons between Complexes I/II and III. - Cytochromes (Heme Proteins) – Contain iron in heme groups and transfer electrons in Complexes III and IV. - This stepwise transfer of electrons generates a proton gradient across the inner mitochondrial membrane, which powers ATP synthesis through oxidative phosphorylation. Complex 1 in ETC: - Complex I (NADH-Q Oxidoreductase) is the first entry point for electrons from NADH in the Electron Transport Chain (ETC). Since NADH donates a pair of electrons (2e⁻) but most carriers can only transfer one electron at a time, FMN (flavin mononucleotide) acts as a transducer, temporarily holding both electrons before passing them one at a time to iron-sulfur (Fe-S) clusters in the complex. Electron Transfer Steps in Complex I: - NADH donates 2e⁻ → FMN picks up both electrons. - FMN passes electrons one at a time → Fe-S clusters. - Fe-S clusters transfer electrons to Coenzyme Q (CoQ/Ubiquinone) → Forms QH₂ (ubiquinol), a fully reduced mobile electron carrier. Proton Pumping and Energy Generation: - As 2e⁻ from NADH move through Complex I, 4 protons (H⁺) are pumped from the matrix to the intermembrane space. - This contributes to the proton-motive force, which later drives ATP synthesis in oxidative phosphorylation. - Summary: Complex I receives electrons from NADH, transfers them through FMN and Fe-S centers to CoQ (forming QH₂), and simultaneously pumps 4 protons across the membrane to help power ATP production. Complex 2- Entry for FADH2: - Complex II (Succinate-Q Reductase) serves as the entry point for electrons from FADH₂ into the Electron Transport Chain (ETC). Unlike Complex I, Complex II is not a proton pump, meaning it does not contribute directly to the proton gradient, which is why FADH₂ generates less ATP than NADH. Electron Transfer Steps in Complex II: - FADH₂ donates 2e⁻ → Electrons are transferred one at a time to Fe-S clusters. - Fe-S clusters pass electrons to Coenzyme Q (CoQ/Ubiquinone) → Forms QH₂ (ubiquinol), a mobile electron carrier. - Key Differences from Complex I: - Complex II is part of the Citric Acid Cycle → Specifically, it contains succinate dehydrogenase, which converts succinate to fumarate, generating FADH₂. - No proton pumping → Unlike Complex I, Complex II does not move protons (H⁺) across the inner mitochondrial membrane, making FADH₂ a less efficient contributor to ATP synthesis. - Summary: Complex II receives electrons from FADH₂, transfers them through Fe-S clusters to CoQ (forming QH₂), but does not pump protons, making it less effective in generating ATP compared to NADH. Complex 3: - Complex III (Q-Cytochrome c Oxidoreductase) is responsible for transferring electrons from QH₂ (ubiquinol) to cytochrome c (Cyt c). However, since QH₂ carries two electrons, while cytochrome c can only carry one, the Q cycle is used to efficiently transfer electrons while maintaining the proton gradient. Electron Transfer Steps in the Q Cycle: - QH₂ donates 2 electrons: - One electron is transferred to cytochrome c (Cyt c). - The second electron is sent back to regenerate another CoQ molecule, which will later become QH₂ again. - Four protons (H⁺) are pumped into the intermembrane space, contributing to the proton gradient. - Cytochrome c (Cyt c) is a mobile carrier: It moves between Complex III and Complex IV, carrying one electron at a time to continue the process. - Summary: The Q cycle allows Complex III to transfer electrons from QH₂ to cytochrome c while pumping 4 protons into the intermembrane space, helping to power ATP synthesis. Proton Gradient: - Generated by oxidation of NADH and FADH2 is called proton-motive force, which powers the synthesis of ATP Chemiosmotic Hypothesis: - Complex IV (Cytochrome c Oxidase) is the final step of the Electron Transport Chain (ETC), where electrons from cytochrome c (Cyt c) are transferred to molecular oxygen (O₂), the final electron acceptor. This process reduces O₂ to water (H₂O) while helping maintain the proton gradient needed for ATP synthesis. - Steps in Complex IV: - Cytochrome c donates electrons to Complex IV, where they pass through copper (Cu) and heme groups. - Four electrons (from four Cyt c molecules) are required to fully reduce one O₂ molecule to two water (H₂O) molecules. - Four protons (H⁺) are pumped from the mitochondrial matrix into the intermembrane space, further strengthening the proton-motive force that drives ATP synthesis. - Summary: Complex IV transfers electrons from cytochrome c to oxygen, forming water and pumping protons into the intermembrane space, playing a crucial role in maintaining the proton gradient for ATP production. Chemiosmotic Hypothesis: - The chemiosmotic hypothesis describes how ATP is produced in mitochondria, bacteria, and chloroplasts. - It explains how the energy from the electron transport chain is used to create ATP. - The hypothesis states that the energy from NADH and FADH2 is used to create an electrochemical gradient across the inner membrane of mitochondria. - This gradient drives the synthesis of ATP. - The process involves the transfer of electrons along the electron transport chain and the exchange of protons across the membrane. The complete oxidation of glucose yields about 30 molecules of ATP through several metabolic processes: 1. Oxidative phosphorylation contributes significantly to ATP production, accounting for about 26 molecules of ATP. This occurs via the electron transport chain (ETC) and ATP synthase, where high-energy electrons from NADH and FADH₂ (produced in the TCA cycle) are used to generate a proton gradient, driving the synthesis of ATP. 2. In glycolysis, glucose is broken down into two molecules of pyruvate, generating 2 ATP directly. 3. The TCA cycle (also known as the citric acid cycle) then processes the pyruvate, generating another 2 ATP directly through substrate-level phosphorylation, while also producing high-energy electron carriers NADH and FADH₂. 4. These NADH and FADH₂ carry high-energy electrons that are passed through the respiratory chain (ETC), leading to the generation of a proton gradient that powers ATP synthesis via oxidative phosphorylation. ATP Synthase & Protein Conducting Unit and Catalytic Unit: - The proton-motive force (PMF), generated by the electron transport chain (ETC), is used to produce ATP through ATP synthase, a large enzyme complex consisting of two major components: F0 and F1. - F0 Component: The F0 component is embedded in the inner mitochondrial membrane and forms the proton channel. Protons (H⁺) move through this channel, driven by the proton-motive force generated by the ETC. This movement causes the F0 subunit to rotate. - F1 Component: The F1 component protrudes into the mitochondrial matrix and contains the catalytic activity of ATP synthase. It is made up of three β subunits, each of which has an active site that can bind to ADP and inorganic phosphate (Pi) to form ATP. - Role of the γ Subunit: The γ subunit connects the F0 and F1 components. As protons flow through the F0 channel, the F0 subunit rotates, causing the γ subunit to spin. This rotational movement induces conformational changes in the β subunits of F1, which allow them to cycle between different states—each state either binding ADP and Pi, catalyzing ATP synthesis, or releasing ATP. - ATP Production: As the γ subunit rotates, it drives ATP synthesis by catalyzing the binding of ADP and Pi to form ATP. The proton gradient is thus converted into the high phosphoryl-transfer potential of ATP, which can be used for cellular energy. - Summary: The proton-motive force powers the rotation of the F0 subunit, which in turn rotates the γ subunit. This movement induces conformational changes in the β subunits of F1, driving the synthesis of ATP from ADP and Pi. Proton Flow Around C ring: - Proton Entry: The A subunit of the ATP synthase complex has two half-channels: one opens to the intermembrane space, and the other opens to the matrix. Protons (H⁺) from the intermembrane space enter the half-channel and bind to a glutamate residue on the c ring. - Proton Movement: Once a proton binds to the glutamate residue, it moves around the c ring and eventually exits through the half-channel that faces the matrix. This movement is powered by the proton gradient between the intermembrane space and the matrix. - Rotation of the c Ring: As protons flow through the c ring, they cause it to rotate. This rotational movement is crucial for ATP synthesis. - Movement of the γ Subunit: The rotation of the c ring drives the movement of the γ subunit, which is connected to both the F0 and F1 components of ATP synthase. The movement of the γ subunit leads to conformational changes in the β subunits of the F1 component, which are responsible for catalyzing ATP synthesis. - Summary: The proton gradient drives protons through the half-channels in the A subunit, causing the c ring to rotate. This rotation moves the γ subunit, which then triggers ATP synthesis in the β subunits of the F1 component of ATP synthases. Proton Flow Through ATP Synthase Leads to the Release of Tightly Bound ATP: - The binding change mechanism explains how ATP synthase synthesizes ATP in response to proton flow. The F1 component of ATP synthase has three β subunits, each of which can exist in one of three distinct conformations: - Tight (T) Form: In this conformation, ATP is synthesized from ADP and inorganic phosphate (Pi). The active site is "tight," making ATP formation energetically favorable and driving the reaction forward. - Open (O) Form: In this state, the β subunit is in a more relaxed conformation, allowing nucleotides (ATP, ADP, and Pi) to bind or be released from the active site. This is when ATP is released from the enzyme after it is synthesized. - Loose (L) Form: In this state, the nucleotides (ADP and Pi) are trapped in the active site. The loose conformation facilitates the binding of ADP and Pi, preparing the enzyme for ATP synthesis. Roles of Glycerol Phosphate and Malate-Aspartate Shuttles Glycerol Phosphate Shuttle: This shuttle helps transport the electrons from cytoplasmic NADH into the mitochondria. In this shuttle, NADH from the cytoplasm donates electrons to dihydroxyacetone phosphate (DHAP), converting it into glycerol 3-phosphate. Glycerol 3-phosphate then passes the electrons to FAD, which is embedded in the inner mitochondrial membrane as part of the glycerol 3-phosphate dehydrogenase enzyme. This FADH₂ donates the electrons to ubiquinone (CoQ) in the electron transport chain, bypassing NADH dehydrogenase (Complex I). This results in the formation of FADH₂, which contributes less ATP than NADH. Malate-Aspartate Shuttle: In this shuttle, electrons from cytoplasmic NADH are transferred to oxaloacetate, forming malate, which can cross the mitochondrial membrane. Once inside the mitochondrion, malate donates electrons to NAD+, converting it back to oxaloacetate and producing NADH. This NADH can now enter the electron transport chain at Complex I, generating a higher ATP yield compared to the glycerol phosphate shuttle. 2. Net ATP Yield from the Complete Oxidation of Glucose Glycolysis: The breakdown of glucose into 2 molecules of pyruvate in the cytoplasm yields 2 ATP and 2 NADH (via the reduction of NAD+). However, since NADH in the cytoplasm cannot directly enter the mitochondria, the shuttle systems are required to transport electrons into the mitochondrion. Malate-Aspartate Shuttle: Each NADH from glycolysis is transported into the mitochondrion and results in the generation of 2.5 ATP per NADH molecule, so the total ATP from the two NADH molecules produced in glycolysis would be 5 ATP. Citric Acid Cycle: Each pyruvate is oxidized to 3 NADH, 1 FADH₂, and 1 GTP (equivalent to ATP). Since there are two pyruvates, the cycle yields 6 NADH, 2 FADH₂, and 2 ATP. Electron Transport Chain (ETC): The NADH produces 2.5 ATP per molecule, and FADH₂ produces 1.5 ATP. This results in: ○ 6 NADH → 15 ATP ○ 2 FADH₂ → 3 ATP Total ATP from the complete oxidation of glucose: ○ 2 ATP from glycolysis ○ 15 ATP from NADH (from TCA cycle) ○ 3 ATP from FADH₂ (from TCA cycle) ○ 2 ATP from GTP (TCA cycle) Estimated Net Yield: 30–32 ATP (depending on the shuttle used). 3. Effect of Uncouplers on Oxidative Phosphorylation Uncouplers are substances that disrupt the proton gradient across the inner mitochondrial membrane, preventing the proton gradient from being used by ATP synthase to produce ATP. This leads to a decrease in ATP production despite continued electron transport. Instead of driving ATP synthesis, the energy from the electrons is released as heat, which can contribute to thermogenesis. Thermogenesis: Some uncouplers are used in regulated processes like non-shivering thermogenesis. In organisms like brown adipose tissue in mammals, uncoupling proteins (UCPs) allow protons to flow back into the mitochondrial matrix without generating ATP. This generates heat, which helps regulate body temperature in cold environments. 4. Compounds that Block Electron Transport and Their Sites of Action Rotenone: Blocks Complex I (NADH-Q oxidoreductase) by preventing the transfer of electrons from NADH to ubiquinone. Antimycin A: Inhibits Complex III (cytochrome bc1 complex) by blocking the transfer of electrons from ubiquinol to cytochrome c. Cyanide, Azide, and Carbon Monoxide: These block Complex IV (cytochrome c oxidase) by inhibiting the transfer of electrons to oxygen, preventing the reduction of oxygen to water. Oligomycin: Inhibits ATP synthase (Complex V) by binding to the F0 subunit, blocking proton flow and preventing ATP synthesis. Summary: Uncouplers reduce ATP production by allowing protons to bypass ATP synthase, leading to heat generation. Specific inhibitors, such as rotenone, antimycin A, and cyanide, block electron transport at different complexes in the electron transport chain, preventing normal electron flow and oxidative phosphorylation.