Gluconeogenesis PDF
Document Details
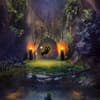
Uploaded by iiScholar
Arizona State University
Tags
Related
- Metabolism II - Biochemistry PDF
- Glucose Tests, Gluconeogenesis, Pentose Phosphate Pathway, & Other CHO Metabolism PDF
- Glucose Tests, Gluconeogenesis, & Pentose Phosphate Pathway PDF
- Carbohydrates PDF - Biochemistry & Molecular Biology
- Glycogen Metabolism and Gluconeogenesis PDF
- Biochemistry or Metabolism (CC-104) Past Paper 2022 PDF
Summary
This document explains the process of gluconeogenesis, a metabolic pathway that synthesizes glucose from non-carbohydrate precursors. It discusses the bypass reactions used to circumvent irreversible steps in glycolysis. The document also details the regulation of glucose metabolism. Learn about the biochemical processes of gluconeogenesis.
Full Transcript
# Gluconeogenesis ## Introduction Lesson 11.1 discusses the process of glycolysis, which catabolizes (i.e., breaks down) glucose into two pyruvate molecules while liberating energy in the form of two net ATP and two NADH. This lesson begins with a discussion of gluconeogenesis, the process by whi...
# Gluconeogenesis ## Introduction Lesson 11.1 discusses the process of glycolysis, which catabolizes (i.e., breaks down) glucose into two pyruvate molecules while liberating energy in the form of two net ATP and two NADH. This lesson begins with a discussion of gluconeogenesis, the process by which cells build glucose from two pyruvate molecules. In addition to the two pyruvate molecules, gluconeogenesis consumes two NADH and six ATP equivalents, meaning it costs more energy to make glucose than is released by glycolysis. Although gluconeogenesis is useful to provide glucose during times of fasting or to recycle glycolysis end products, gluconeogenesis and glycolysis must be regulated to prevent the wasting of energy. In addition to the process of gluconeogenesis, this lesson also discusses the regulation of glucose metabolism. ## 11.2.01 Gluconeogenesis Gluconeogenesis builds up glucose from two pyruvate molecules. Unlike glycolysis, which can occur in the cytosol of all cells, gluconeogenesis in mammals mainly occurs in the liver, although a few other cell types can support gluconeogenesis in limited amounts. This compartmentalization of processes reflects the liver's role in providing fuel, such as glucose, for the rest of the body during times of fasting. Although glycolysis and gluconeogenesis are highly related and share several enzymes, gluconeogenesis is not simply glycolysis in reverse. Glycolysis uses three biochemically irreversible enzyme-catalyzed reactions, namely the reactions catalyzed by hexokinase, phosphofructokinase-1, and pyruvate kinase (see Concept 11.1.01 and Concept 11.1.02). Although glycolysis uses these enzymes in the catabolism of glucose, gluconeogenesis cannot use the same enzymes in the anabolism of two pyruvate molecules back to glucose. Instead, for each of these three irreversible steps of glycolysis, the process of gluconeogenesis must use one or more bypass reactions, which are themselves irreversible and are each catalyzed by unique enzymes not used in glycolysis. Figure 11.25 depicts an overview of glycolysis with its irreversible enzymes and the bypass reactions used in gluconeogenesis. The other seven enzymes of glycolysis, which catalyze reversible reactions, are shared with gluconeogenesis. ### Figure 11.25: The irreversible enzymes of glycolysis and gluconeogenesis. The net reaction for gluconeogenesis is: $2$ Pyruvate + $6$ ATP + $2$ NADH + $2$ H+ → Glucose + $6$ ADP + $6$ Pi + $2$ NAD+ ## Gluconeogenesis: The First Set of Bypass Reactions Because glycolysis ends with the production of pyruvate, this discussion of gluconeogenesis begins with pyruvate. However, it is worth noting that other starting points are possible and several of these are discussed in Concept 11.2.02. Pyruvate is produced by the final enzyme of glycolysis, pyruvate kinase, which transfers a phosphate from phosphoenolpyruvate to ADP, thereby forming pyruvate and ATP. Because the pyruvate kinase reaction is biochemically irreversible, pyruvate kinase cannot convert pyruvate back to phosphoenolpyruvate for gluconeogenesis. Instead, this single reaction of glycolysis requires two bypass reactions and therefore two enzymes to convert pyruvate to phosphoenolpyruvate in gluconeogenesis. The first reaction is the carboxylation of pyruvate to form oxaloacetate, catalyzed by the enzyme pyruvate carboxylase. The carboxyl group comes from a bicarbonate ion, which itself comes from the reaction carbon dioxide with water. The pyruvate carboxylase reaction consumes on molecule of ATP per molecule of pyruvate. Unlike glycolysis, in which every enzyme is cytosolic, pyruvate carboxylase is a mitochondrial enzyme. Therefore, the pyruvate molecule must first be transported to the mitochondria before gluconeogenesis can occur. The pyruvate carboxylase reaction is shown in Figure 11.26. ### Figure 11.26: The pyruvate carboxylase reaction, the first of two reactions needed to bypass the pyruvate kinase reaction. The second bypass reaction is catalyzed by the enzyme phosphoenolpyruvate carboxykinase (PEPCK). In gluconeogenesis, the PEPCK reaction accomplishes two sub-reactions: first, the oxaloacetate is decarboxylated to form an enolate; second, a phosphate is transferred from GTP to the substrate to form phosphoenolpyruvate. The same carboxyl group that was added by pyruvate carboxylase is removed by PEPCK. Humans possess both a mitochondrial and a cytosolic isoform of PEPCK. Therefore, the PEPCK reaction can occur in either compartment, depending on the levels of other metabolites and other metabolic needs (see Concept 11.2.02). The PEPCK reaction is shown in Figure 11.27. ### Figure 11.27: The phosphoenolpyruvate carboxykinase (PEPCK) reaction. Importantly, although the pyruvate kinase reaction produces one ATP molecule per pyruvate formed, the bypass reactions together consume two ATP equivalents (one ATP and one GTP) to convert one pyruvate to phosphoenolpyruvate. If both glycolysis and gluconeogenesis were active at the same time, pyruvate and phosphoenolpyruvate would cycle between the two processes, resulting in a futile cycle. Because the net effect of a futile cycle is simply to hydrolyze ATP (i.e., no other metabolite levels are changed), futile cycles are said to "waste" energy. To prevent wasting of ATP, the irreversible enzymes of glycolysis and gluconeogenesis are tightly regulated, as discussed in Concept 11.2.04. ## Gluconeogenesis: Most Enzymes Are Shared with Glycolysis After phosphoenolpyruvate is synthesized by PEPCK, it is trafficked to the cytosol if not already there. At this point, the next six reactions are catalyzed by the same enzymes used in glycolysis. This can occur because these enzymes all catalyze reversible reactions and therefore can operate in either direction based on the relative concentrations of the reactants and products. Notably, 3-phosphoglycerate is phosphorylated to from 1,3-bisphosphoglycerate during this process. This reaction, catalyzed by phosphoglycerate kinase, consumes one molecule of ATP per reaction. 1,3-Bisphosphoglycerate is then reduced by glyceraldehyde-3-phosphate dehydrogenase, consuming one NADH and producing one molecule of glyceraldehyde 3-phosphate (G3P). At this point one pyruvate molecule has been converted to one G3P molecule, and 3 ATP equivalents and one NADH have been consumed. To build a full glucose molecule, each of the previous steps must occur twice, consuming a total of 6 ATP equivalents, 2 NADH, and 2 pyruvates to make two G3P molecules. One of the G3P molecules is isomerized to dihydroxyacetone phosphate (DHAP) by triose phosphate isomerase. DHAP and the remaining molecule of G3P then combine to make fructose 1,6-bisphosphate in a reaction catalyzed by aldolase. ## Gluconeogenesis: The Final Pair of Bypass Reactions In glycolysis, fructose 1,6-bisphosphate is synthesized in an irreversible reaction by the enzyme phosphofructokinase-1. In gluconeogenesis, the enzyme fructose-1,6-bisphosphatase (FBPase-1) catalyzes the bypass reaction. Whereas the glycolysis reaction is a phosphate transfer reaction that consumes an ATP molecule, the bypass reaction in gluconeogenesis is a hydrolysis reaction that does not involve ATP at all. In this reaction, the phosphate on carbon 1 is hydrolyzed, producing fructose 6-phosphate and inorganic phosphate (Pi, Figure 11.28). ### Figure 11.28: The fructose-1,6-bisphosphatase reaction of gluconeogenesis is used to bypass the phosphofructokinase-1 reaction of glycolysis. Fructose 6-phosphate is then isomerized to glucose 6-phosphate by the same reversible enzyme used in glycolysis, phosphoglucose isomerase. The final step of gluconeogenesis is another bypass reaction to oppose the irreversible reaction catalyzed by hexokinase. The bypass reaction is catalyzed by glucose-6-phosphatase, as shown in Figure 11.29. ### Figure 11.29: The glucose-6-phosphatase reaction of gluconeogenesis bypasses the hexokinase reaction of glycolysis. Without the phosphate group, the free glucose molecules are no longer trapped in the cell. As gluconeogenesis proceeds, the intracellular glucose concentration in the liver cell eventually surpasses the blood glucose concentration level and glucose passively leaves the liver cell by facilitated diffusion through glucose transporters. The glucose molecules are then carried through the blood to target tissues. The enzymes that differ between glycolysis and gluconeogenesis are summarized in Table 11.1. ### Table 11.1: Irreversible glycolysis enzymes and corresponding bypass enzymes of gluconeogenesis. Gluconeogenesis steps are numbered starting from pyruvate. | Step of Glycolysis | Enzyme of Glycolysis | Corresponding enzyme(s) of Gluconeogenesis | Step of Gluconeogenesis | |:---:|:---:|:---:|:---:| | 1 | Hexokinase | Glucose-6-phosphatase | 11 | | 3 | Phosphofructokinase-1 | Fructose-1,6-bisphosphatase | 9 | | 10 | Pyruvate kinase | Phosphoenolpyruvate carboxykinase (PEPCK) | 2 | | | | Pyruvate carboxylase | 1 | ## Concept Check 11.2 One molecule of glucose is broken down through glycolysis. The resulting pyruvate molecules are then immediately sent through gluconeogenesis to rebuild glucose. What is the net outcome of these processes? **Solution:** ## 11.2.02 Alternate Entry Points of Gluconeogenesis This discussion of gluconeogenesis so far focused on the production of glucose from pyruvate molecules. However, many other molecules can also be used as starting substrates for gluconeogenesis. For example, alanine can be converted to pyruvate through deamination. Lactate can similarly enter gluconeogenesis. As discussed in Concept 11.1.04, pyruvate is reversibly reduced to lactate during the process of lactic acid fermentation, which occurs in oxygen-poor (or mitochondria-lacking) tissue. In the oxygen-rich liver, lactate can be oxidized back into pyruvate, which can then enter gluconeogenesis. In addition to molecules that are converted to pyruvate, many gluconeogenic substrates are metabolized directly into oxaloacetate without becoming pyruvate first. Although oxaloacetate appears only after the first step of the classical gluconeogenesis pathway, substrates that can be metabolized directly to oxaloacetate can bypass the pyruvate carboxylase step and therefore consume less ATP. In addition, because oxaloacetate is also a member of the citric acid cycle (see Chapter 12), molecules that are metabolized into other citric acid cycle members can be further metabolized into oxaloacetate to act as a gluconeogenic substrate. Examples of molecules that produce oxalactetate-either directly or through the citric acid cycle-include many amino acids and odd-chain fatty acids. The conversion of these molecules into gluconeogenic precursors is shown in Figure 11.30. For details of the biochemical pathways involved in producing these gluconeogenic precursors, see Chapters 12 and 13. Notably, acetyl-CoA cannot act as a precursor for gluconeogenesis. ### Figure 11.30: Many molecules can server as gluconeogenesis substrates by being converted to oxaloacetate. As discussed in Concept 11.2.01, oxaloacetate can be converted to phosphoenolpyruvate (PEP) by a cytosolic isoform of PEPCK. To enter the cytosol, however, oxaloacetate must first be reduced to malate in the mitochondrial matrix and re-oxidized to oxaloacetate in the cytosol. Like the malate-aspartate shuttle (discussed in Chapter 12), this process also results in the effective transport of NADH from the mitochondria to the cytosol, and the cytosolic NADH can then be used to power the GAPDH reaction. Interestingly, the oxidation of lactate to pyruvate produces cytosolic NADH directly. In this case, the malate shuttle is no longer needed to transport NADH, so the mitochondrial isoform of PEPCK can be used to convert oxaloacetate to phosphoenolpyruvate (PEP). PEP can then be exported to the cytosol directly to proceed with gluconeogenesis. The difference in NADH production, and consequently the difference in PEPCK isoform localization, is shown in Figure 11.31. ### Figure 11.31: In humans, the choice of PEPCK isoform during gluconeogenesis is influenced by the need to shuttle NADH to the cytosol. Glycerol, produced as a byproduct of triglyceride catabolism, can also serve as a gluconeogenic precursor. Glycerol can be phosphorylated into glycerol 3-phosphate before being oxidized to dihydroxyacetone phosphate (DHAP). DHAP can then enter gluconeogenesis directly as one of the metabolites found along the pathway. In the fasting state, the liver breaks down fat to power its ability to provide glucose for the rest of the body. Therefore, the glycerol backbones of triglycerides produced by this process commonly proceed through gluconeogenesis rather than glycolysis. ## 11.2.03 The Cori Cycle The Cori cycle is an example of coordination across tissues and organ systems that allows multicellular organisms to adapt and thrive in a variety of habitats and conditions. Whereas most cells in aerobic organisms can use aerobic respiration to provide ample ATP production (see Chapter 12), cells without mitochondria (eg, red blood cells) and hypoxic cells (eg, vigorously contracting muscle) are limited to glycolysis for energy production. Without aerobic respiration, fermentation is required to convert the NADH product of glycolysis back into NAD+, which can then be used to power further rounds of glycolysis (see Concept 11.1.04). Unlike the volatile ethanol and CO2 produced during alcoholic fermentation, the lactate produced during lactic acid fermentation remains in solution. The cells that produce lactate can export it and, in multicellular organisms, send it through the blood to the oxygen-rich liver, which can use aerobic respiration to produce an abundant supply of NAD+. Therefore, the liver can oxidize lactate back to pyruvate, which can then be metabolized further. The pyruvate molecules in the liver undergo gluconeogenesis to synthesize new glucose molecules (Concept 11.2.01). These new glucose molecules are then exported from the liver through the blood to the target tissues that need glucose. An overview of the Cori cycle is shown in Figure 11.32. ### Figure 11.32: The Cori cycle. Although cycling between glycolysis and gluconeogenesis within a single cell would be a wasteful futile cycle, the separation of processes across different tissues is an efficient means of resource management by multicellular organisms. It costs the liver more ATP to synthesize glucose than can be produced from glycolysis, but the oxygen-rich liver has alternate sources of energy that produce much higher ATP yields (eg, aerobic respiration of stored glycogen and fat). Red blood cells and hypoxic muscle, on the other hand, are dependent on anaerobic glycolysis for ATP production. Rather than allow those tissues to run out of glucose, the liver spends ATP to power gluconeogenesis and to provide the whole body with a consistent supply of fuel molecules. ## 11.2.04 Regulation of Glucose Metabolism Glycolysis, like most metabolic pathways, must be regulated to maintain relatively constant metabolite levels. Levels of ATP that are too high, for example, may shift the equilibrium of ATP-consuming reactions, whereas levels that are too low would not allow energy-requiring coupled reactions to occur. In tissues that express gluconeogenesis enzymes (eg, liver), the regulation of glycolysis must be even more controlled to prevent the occurrence of a futile cycle. This concept provides an overview of the regulation of glycolysis and gluconeogenesis. ### Allosteric Feedback Mechanisms Regulate Glycolytic Flux In tissues that do not perform gluconeogenesis (eg, muscle, red blood cells), the flux-or overall rate of glycolysis is largely controlled by feedback inhibition. In other words, excessive production of products causes the rate of glycolysis to slow down, whereas low levels of products allow glycolysis to speed up. As discussed in Lesson 6.1, these regulatory mechanisms are focused mainly on the enzymes that catalyze irreversible reactions, namely the enzymes hexokinase, phosphofructokinase-1, and pyruvate kinase. In most cells, hexokinase, which catalyzes the first step of glycolysis, is inhibited by its product, glucose 6-phosphate. Glucokinase, the hexokinase isoform in the liver and some other tissues, lacks this regulation so that it can serve as a glucose sensor. In other words, high levels of glucose result in high levels of glucose 6-phosphate in the liver so that the liver can "sense" the blood glucose level, whereas other tissues limit glucose 6-phosphate production to the amount those tissues need for their own metabolism. Pyruvate kinase, which catalyzes the final step of glycolysis, is allosterically inhibited by its product (ATP), as well as by acetyl-CoA and long-chain fatty acids. Acetyl-CoA is a downstream metabolite of glucose catabolism (see Lesson 12.1). Long-chain free fatty acids are an alternate energy source for tissues such as muscle, and their presence indicates glycolysis is not needed for energy. These mechanisms are summarized in Figure 11.33. ### Figure 11.33: Allosteric regulatory mechanisms of hexokinase and pyruvate kinase Phosphofructokinase-1 (PFK-1) catalyzes the third step of glycolysis. This reaction is also the earliest committed step of glycolysis (see Concept 11.1.01); therefore, PFK-1 is the most highly regulated enzyme of glycolysis. Like hexokinase and pyruvate kinase, PFK-1 is affected by feedback inhibition. In this case, the allosteric inhibitor is ATP, which is a product of the glycolysis pathway rather than a product of the reaction catalyzed by PFK-1. PFK-1 can also be activated by the molecules ADP and AMP. Therefore, when ATP levels drop (and ADP and AMP levels rise), not only is feedback inhibition relieved, but enzyme activity is also stimulated to facilitate more ATP production (Figure 11.34). ### Figure 11.34: ATP decreases PFK-1 activity by feedback inhibition. High levels of ADP or AMP stimulate PFK-1 activity by feedback activation. In addition, PFK-1 is regulated by the levels of the metabolite citrate. Citrate is a member of the citric acid cycle, which occurs in the mitochondrial matrix, whereas PFK-1 (like the other enzymes of glycolysis) is cytosolic. However, citrate is trafficked to the cytosol during the process of fatty acid synthesis (see Concept 13.1.04), which occurs during periods of energy excess. Therefore, citrate inhibits PFK-1 because its presence in the cytosol is a sign that glycolysis does not need to occur to meet a cell's energy needs. One of the most potent allosteric stimulators of PFK-1 activity is the molecules fructose 2,6-bisphosphate (F2,6BP). F2,6BP is synthesized by phosphorylation of fructose 6-phosphate by the enzyme phosphofructokinase-2 (PFK-2) and is degraded back to fructose 6-phosphate by the enzyme fructose-2,6-bisphosphatase (FBPase-2). Note that the position of the phosphate group on the molecules is very important in distinguishing the molecules F2,6BP, PFK-2, and FBPase-2 are involved in regulating glycolysis (and gluconeogenesis), whereas F1,6BP, PFK-1 and FBPase-1 are direct members of glycolysis (or gluconeogenesis, Figure 11.35). ### Figure 11.35: Fructose 2,6-bisphosphate, PFK-2, and FBPase-2 all regulate glycolysis (and gluconeogenesis) and are not to be confused with fructose 1,6-bisphosphate, PFK-1, or FBPase-1. F2,6BP and its associated enzymes are especially important in the liver, where hormones induce covalent regulation of PFK-2/FBPase-2 to help coordinate glycolysis and gluconeogenesis. However, F2,6BP also plays an important role in regulating glycolysis in nongluconeogenic tissues, such as muscle. In muscle, PFK-2 and F2,6BP levels are regulated by substrate levels (ie, fructose 6-phosphate concentration) rather than covalent modifications to the enzyme. To understand the role of F2,6BP in glycolysis regulation, consider a muscle cell that has received a signal that causes a sudden increase in glucose 6-phosphate levels. Phosphoglucose isomerase, which is reversible and unregulated, converts glucose 6-phosphate to fructose 6-phosphate (F6P); however, PFK-1 activity may not be able to keep up with the sudden influx of F6P. The accumulating F6P then proceeds down a side pathway, catalyzed by PFK-2, to make F2,6BP. F2,6BP then plays its role as a potent allosteric activator of PFK-1 and therefore of glycolysis, which relieves the buildup of F6P and allows the cell to come back to homeostasis. This scenario is depicted in Figure 11.36. ### Figure 11.36: In muscle, a buildup of fructose 6-phosphate leads to production of fructose 2,6-bisphosphate. Fructose 2,6-bisphosphate then activates PFK-1 to stimulate glycolysis. A summary of the regulatory mechanisms controlling glycolysis in nongluconeogenic cells is given in Table 11.2. ### Table 11.2: Glycolysis regulatory mechanisms in nongluconeogenic tissue | Enzyme | Inhibitor | Activator | Rationale for regulation | |:---:|:---:|:---:|:---:| | Hexokinase | Glucose 6-phosphate | | Feedback inhibition | | | Citrate | | Sign of fatty acid synthesis | | Phosphofructokinase-1 | ATP | ADP | Feedback inhibition | | | | AMP | Sign of low energy stores | | | | Fructose 2,6-bisphosphate | Sign of low energy stores | | | | | Sign of slow flux | | Pyruvate kinase | ATP | Fructose 1,6-bisphosphate | Feedforward activation | | | Acetyl-CoA | | Feedback inhibition | | | Long-chain fatty acids | | Feedback inhibition | | | | | Alternate energy source | ### Covalent Regulation Affects Glycolysis in the Liver The liver, which must coordinate both glycolysis and gluconeogenesis together, has more complex regulatory mechanisms that allow for the control of both processes. Because the liver must take into account signals from other tissues and organs, much of liver glycolysis regulation is controlled by the action of hormones that induce covalent regulation on certain enzymes. Two important hormones in the regulation of glycolysis and gluconeogenesis are insulin and glucagon. Insulin is a peptide hormone released when blood glucose is high (eg, during the fed state), and it binds to the insulin receptor. In contrast, the peptide hormone glucagon is released when blood glucose is low (eg, during the fasting state), and it binds to the glucagon receptor. Epinephrine (also known as adrenaline) is another hormone that binds to a Gsa-coupled G protein-coupled receptor (GPCR) and has similar action to glucagon. Both insulin and glucagon have many and varied effects. One of the most prominent effects of glucagon is the eventual activation of cAMP-dependent protein kinase A (PKA), which phosphorylates many enzymes involved in glycolysis and gluconeogenesis. In the context of glycolysis and gluconeogenesis, insulin activates phosphoprotein phosphatase-1 (PP1), which dephosphorylates the enzymes acted upon by PKA (Figure 11.37). ### Figure 11.37: Glucagon binding results in the phosphorylation of many enzymes involved in energy metabolism. Insulin binding results in the dephosphorylation of many of those same enzymes. Although glucose is the preferred fuel for most cells, high levels of blood glucose are damaging. Consequently, insulin signals for many tissues to take up glucose and signals the liver to upregulate glycolysis to decrease blood glucose levels. To do so, insulin triggers the dephosphorylation of PFK-2. Dephosphorylation of PFK-2 activates the enzyme, resulting in an increase in liver F2,6BP levels. As discussed in the previous section, F2,6BP is a potent allosteric activator of PFK-1 and therefore of glycolysis. Because the liver can also perform gluconeogenesis, insulin must also inhibit gluconeogenesis to prevent a futile cycle. Not only does F2,6BP stimulate PFK-1, but it also inhibits FBPase-1, the bypass enzyme that corresponds to PFK-1 (Figure 11.38). ### Figure 11.38: Insulin dephosphorylates and activates PFK-2, which produces F2,6BP. F2,6BP stimulates glycolysis by activating PFK-1 and inhibits gluconeogenesis by inhibiting FBPase-1. Note that insulin-mediated stimulation of glycolysis (and inhibition of gluconeogenesis) continues despite the liver cell having an excess of energy (ie, high ATP levels). This is because F2,6BP overrides the feedback regulation that would normally inhibit PFK-1 (ie, high [ATP], high [citrate]). To help the liver cell manage the influx of glucose and increased glycolytic flux, insulin also stimulates glycogen synthesis (Lesson 11.4) and fatty acid synthesis (Lesson 13.1). Glucagon, released when blood sugar levels are low, signals the liver to upregulate gluconeogenesis so that it can synthesize and release glucose for the rest of the body. To prevent a futile cycle, glucagon must therefore also inhibit glycolysis in the liver cell. To accomplish both goals, glucagon signaling leads to the stimulation of PKA, which phosphorylates and inactivates the enzyme PFK-2. Interestingly, the enzymes PFK-2 and FBPase-2 exist as two domains on the same protein; therefore, the same event that leads to phosphorylation and inhibition of PFK-2 also causes activation of FBPase-2. This covalent regulation leads to a decrease in F2,6BP levels, increased gluconeogenesis, and decreased glycolysis (Figure 11.39). PKA also phosphorylates pyruvate kinase, inhibiting it and decreasing glycolysis even further. ### Figure 11.39: Glucagon causes the phosphorylation and activation of FBPase-2 to degrade F2,6BP. Low F2,6BP levels promote gluconeogenesis and prevent glycolysis. Glucagon is normally secreted during the fasting state, when stores of readily available fuel for energy are low. Nevertheless, the liver expends any energy it does have to create fuel for the rest of the body. To help the liver cell power gluconeogenesis despite the low stores of fuel in the blood, glucagon also stimulates glycogenolysis (Lesson 11.4) and fatty acid oxidation (Chapter 13). Like glucagon, the hormone epinephrine activates PKA, and therefore also stimulates gluconeogenesis in the liver cell. Yet epinephrine stimulates glycolysis in the muscle cell. This phenomenon illustrates the differing effects a signaling molecule may have on different tissues, depending on the genes and proteins expressed. Liver tissue expresses a phosphorylatable isoform of PFK-2 and therefore downregulates glycolysis upon epinephrine exposure. Muscle cell PFK-2 is not regulated by phosphorylation, and therefore epinephrine-induced increases in fructose 6-phosphate cause an increase in glycolysis