AI BIOS 313 Exam 1 Study Guide PDF
Document Details
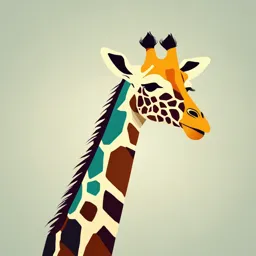
Uploaded by SprightlySard4679
Tags
Summary
This study guide covers the early evidence of microbial life on Earth, focusing on stromatolites and other biosignatures. It explores the formation process and significance of stromatolites, and also examines other evidence for early life, including carbon isotope ratios. The guide also discusses the concept of biosignatures and challenges in interpretation.
Full Transcript
EXAM 1 study guide 1. Early evidence of microbial life on Earth: According to current scientific understanding, the oldest evidence of microbial life on Earth dates back approximately 3.5 billion years, primarily found in fossilized microbial mats within structures called stromatolites, whi...
EXAM 1 study guide 1. Early evidence of microbial life on Earth: According to current scientific understanding, the oldest evidence of microbial life on Earth dates back approximately 3.5 billion years, primarily found in fossilized microbial mats within structures called stromatolites, which are layered rock formations created by the activity of ancient bacteria, mainly cyanobacteria, that trapped sediment as they grew in shallow water environments. Key points about early life on Earth: Stromatolite evidence: The most widely accepted evidence for early life comes from stromatolites, which exhibit distinct layered structures formed by the accumulation of microbial mats over time. These structures are found in ancient rock formations from the Archean Eon, providing a fossil record of microbial activity from around 3.5 billion years ago. Microbial mat composition: These microbial mats were likely composed of communities of photosynthetic bacteria that would trap sediment and minerals as they grew upwards towards light, creating the layered appearance characteristic of stromatolites. Other evidence: While stromatolites are the primary evidence, other lines of research include the study of carbon isotope ratios in ancient rocks, which can indicate the presence of biological activity due to the preferential use of lighter carbon isotopes by living organisms. Challenges in interpretation: Although the evidence points to microbial life around 3.5 billion years ago, some scientists debate the exact interpretation of certain fossil structures, as abiotic processes could sometimes mimic biological features. Life on Earth over Time: Earth formed: 4.6 bya Bacteria/Archaea: ~3.8 bya -Earlier microbes: maybe 4.3 bya Oxygen (O2): ~2.4 bya -21% O2: 500-800 mya Eukaryotes: ~2 bya Mammals: ~200-250 mya Humans: ~300,000 ya 2. What are stromatolites? Stromatolites are layered sedimentary rock formations primarily created by the activity of photosynthetic microorganisms like cyanobacteria, which form microbial mats that trap and bind sediment particles, leading to the gradual accumulation of calcium carbonate layers, resulting in a distinctive layered structure that serves as significant evidence of early life on Earth; essentially, they are "living fossils" built up over time by microbial communities in shallow water environments. Key points about stromatolites: Formation process: Microbial mats: Cyanobacteria and other microorganisms form sticky mats on the seafloor, trapping sediment particles and minerals from the surrounding water. Calcium carbonate precipitation: As the microbial mats grow, they induce the precipitation of calcium carbonate minerals, forming thin layers that build upon each other. Layered structure: This repeated process of sediment trapping and mineral precipitation creates the characteristic layered appearance of stromatolites. Significance as evidence of early life: Ancient fossils: Stromatolites are among the oldest known fossils on Earth, dating back billions of years to the Precambrian era, providing evidence of early life forms. Oxygen production: Early cyanobacteria within stromatolites are believed to have played a crucial role in oxygenating the Earth's atmosphere through photosynthesis. Structure and morphology: Diverse shapes: Stromatolites can form various shapes depending on environmental conditions, including dome-like, columnar, or sheet-like structures. Microscopic layers: The layered structure of a stromatolite can be examined under a microscope to reveal the fossilized remains of the microbial communities that created it. 3. What is a biosignature? Evidence for life in the geological record is called a biosignature. A "biosignature" is any substance, molecule, isotopic ratio, or physical structure that provides scientific evidence of past or present life on a planet. However, it is hard to rule out nonbiogenic explanations. Many potential biosignatures can also be produced by non-biological processes (e.g., volcanic activity can create methane), making it difficult to definitively attribute their origin to life. The most convincing evidence for early microbial life is the visual appearance of microfossils. The earliest microfossils accepted as biogenic are dated at 2.0 Gyr (aka 2 Giga years aka 2 billion years ago). ― Filamentous prokaryotes ― Colonial cyanobacteria Biosignatures are chemical indicators of life. Isotope ratios: ― An isotope ratio provides a biosignature with quantitative evidence, if the ratio between certain stable isotopes of a given element is altered by biological activity. Chemical biosignatures: ― Cyanobacterial hopanoids are steroid-like membrane molecules that are particularly durable. 4. Origin of elements for initial microbial life: The building blocks of early microbial life likely originated from a combination of sources, with the most prominent hypotheses pointing towards chemical reactions in the early Earth's atmosphere and oceans, specifically near hydrothermal vents, as well as the delivery of organic molecules through meteorites impacting the planet; these sources provided the necessary elements like carbon, hydrogen, oxygen, nitrogen, phosphorus, and sulfur, which could have combined under the right conditions to form the basic components of life, such as amino acids and nucleotides. Key potential sources: Hydrothermal vents: Considered a prime candidate due to the extreme heat, pressure, and chemical-rich fluids emanating from them, which could have provided the energy and necessary elements for the formation of complex organic molecules through abiogenesis (the origin of life from non-living matter). Chemical reactions at vents: The interaction between hot vent fluids and the surrounding seawater could have facilitated chemical reactions that produced building blocks like amino acids and nucleotides. Energy source: The chemical gradients present near vents could have served as an energy source for early life forms to utilize through chemosynthesis. Early Earth's atmosphere and oceans: Lightning strikes: Electrical discharges from lightning could have provided the energy needed to break apart simple molecules in the atmosphere, allowing them to recombine into more complex organic compounds. UV radiation: Ultraviolet radiation from the young sun could have driven chemical reactions in the primitive atmosphere, leading to the formation of prebiotic molecules. "Primordial soup": The accumulation of these organic molecules in the early oceans could have created a "primordial soup" where further reactions could occur, leading to the formation of larger, more complex molecules. Meteorites: Extraterrestrial organic compounds: Analysis of meteorites has revealed the presence of amino acids and other organic molecules, suggesting that some building blocks of life could have been delivered to Earth from space. Delivery mechanism: When meteorites impact the Earth, they could have brought these prebiotic molecules into the early Earth's environment. 5. Evidence supporting the RNA world model: From PowerPoint: The RNA world is a model in which RNA performed all the informational and catalytic roles of today’s DNA and proteins. Genome sequences reveal thousands of catalytic and structural RNAs. RNA requires less energy to form and degrade, compared to DNA. Catalytic RNA molecules called ribozymes have been shown to exhibit enzymatic properties analogous to those of proteins. The ribozyme model shows how in early cells, RNA may have fulfilled key functions that are now performed by DNA and proteins. The RNA world hypothesis proposes that, in the early stages of life on Earth, RNA molecules played a central role in both storing genetic information and catalyzing chemical reactions, essentially acting as the primary functional molecule before the emergence of DNA and proteins as we know them today; this is supported by evidence like the catalytic abilities of ribozymes, RNA's crucial role in protein synthesis, and the ability of certain ribozymes to synthesize complementary RNA strands, suggesting self- replication potential. Key points about the RNA world hypothesis: Catalytic RNA (Ribozymes): One of the strongest pieces of evidence is the discovery of ribozymes, RNA molecules with catalytic activity, demonstrating that RNA can act as an enzyme, performing chemical reactions without the need for proteins. Ribosome Structure: The ribosome, the cellular machinery responsible for protein synthesis, contains a large RNA component (rRNA) which acts as the catalytic center, further supporting the idea of RNA's early catalytic role. RNA Self-Replication: Some ribozymes have been shown to be capable of self-replication by catalyzing the synthesis of complementary RNA strands, indicating a potential mechanism for early genetic information propagation. RNA Viruses: The existence of RNA viruses that solely rely on RNA for genetic information and replication further suggests that RNA could have functioned as the primary genetic material in early life. Comparing RNA and DNA: Stability: DNA is generally more stable than RNA due to its double-stranded structure, making it better suited for long-term genetic storage, while RNA's single-stranded nature allows for greater flexibility and potential catalytic activity. Chemical Diversity: RNA contains uracil instead of thymine, which may have provided additional chemical diversity in early evolutionary stages, enabling a wider range of catalytic functions. Why RNA might have been central to early life: Combined Function: RNA's ability to store genetic information and catalyze chemical reactions simultaneously would have been advantageous in a primitive environment where complex protein machinery was not yet developed. Prebiotic Synthesis: Studies have shown that RNA nucleotides can be formed under conditions likely present on early Earth, making it plausible that RNA could have arisen abiogenically. 6. Definition of a clade: A clade, in phylogenetic terms, is a group of organisms that includes a common ancestor and all of its descendants, essentially forming a single branch on a phylogenetic tree, representing a lineage that shares a common evolutionary history; you can identify a clade on a phylogenetic tree by simply "clipping" a single branch, where all organisms on that branch belong to the same clade, signifying they share a common ancestor. Identifying clades on a tree: To identify a clade on a phylogenetic tree, simply look for a branch point (node) and include all the organisms stemming from that node. Evolutionary relationships: Clades help visualize evolutionary relationships between organisms, showing which groups share a more recent common ancestor with each other compared to other groups. Example: Imagine a phylogenetic tree with branches representing mammals, reptiles, and birds. If you "clip" the branch containing mammals, all the organisms on that branch (including humans, whales, and bats) would belong to the "mammal" clade, indicating they all share a common ancestor that was not shared with reptiles or birds. 7. What does "monophyletic group" mean? A "monophyletic group" refers to a group of organisms that includes a common ancestor and all of its descendants, essentially forming a single evolutionary lineage, and is synonymous with a "clade" in evolutionary biology; meaning a clade is always a monophyletic group and vice versa. Key points about monophyletic groups: Definition: A group consisting of a common ancestor and all its descendants. Clade relationship: A monophyletic group is the same as a clade on a phylogenetic tree. Identifying a monophyletic group: You can identify a monophyletic group by "cutting" a single branch on a phylogenetic tree, where the cut branch represents the entire group. Contrast with Paraphyletic and Polyphyletic Groups: A monophyletic group includes a common ancestor and all its descendants, forming a single, unified clade, while a paraphyletic group only includes a common ancestor and some of its descendants, leaving out a related lineage, and a polyphyletic group consists of organisms with different common ancestors, not sharing a single recent evolutionary origin; examples include: Monophyletic group: Mammals (including humans, whales, and bats) - all descend from a single common ancestor with shared characteristics like hair and mammary glands. Paraphyletic group: "Fish" - while many fish share a common ancestor, this group excludes tetrapods (like amphibians, reptiles, birds, and mammals) which also evolved from that same ancestor. Polyphyletic group: "Flying vertebrates" - includes bats and birds, which evolved the ability to fly independently from different ancestors, not sharing a recent common ancestor that could fly. 8. What is reductive evolution? Reductive evolution, also called degenerative evolution, is an evolutionary process where an organism loses genetic information, leading to a simplified genome and often a loss of complex functions, typically occurring when an organism becomes dependent on a host environment and no longer needs certain genes to survive, like in the case of intracellular parasites or endosymbionts; examples include mitochondria and chloroplasts, which are believed to have originated from bacteria that underwent significant genome reduction after becoming incorporated into eukaryotic cells, losing many genes that were no longer necessary due to the host providing essential functions. How it occurs: Gene loss through mutations: When a gene becomes nonfunctional due to mutations and is not selected against because the required function is provided by the host environment, it is likely to be lost over generations due to genetic drift. Horizontal gene transfer: In some cases, genes can be transferred to a host organism, further reducing the need for the symbiont to maintain those genes in its own genome. Relaxed selection: When an organism lives in a stable, nutrient-rich environment provided by a host, there is less selective pressure to maintain genes that are not essential for survival in that niche. Examples of organisms that have undergone reductive evolution: Mitochondria and chloroplasts: These organelles, essential for cellular respiration and photosynthesis respectively, are believed to have originated from free-living bacteria that became endosymbionts within early eukaryotic cells, losing most of their original genetic material over time. Bacterial endosymbionts: Many bacteria that live inside the bodies of insects or other organisms have significantly reduced genomes compared to their free-living relatives, as they rely on their host for many nutrients and functions. Parasites: Obligate parasites, like tapeworms, often lack complex digestive systems because they absorb nutrients directly from their host, leading to the loss of genes related to digestion. Selective pressures driving reductive evolution: Energy conservation: Maintaining a large genome requires energy, so in environments where resources are limited, selection can favor organisms with smaller genomes that require less energy to replicate. Adaptation to a specialized niche: When an organism becomes highly specialized to live within a host, genes that are no longer needed for that specific environment can be lost. Reduced competition with the host: By losing functions that are already provided by the host, a symbiont can avoid potential conflicts and optimize its relationship with the host. 9. Traits common to all cells: All cells share fundamental characteristics including a plasma membrane, cytoplasm, ribosomes, DNA as their genetic material, and basic metabolic processes, which strongly supports the theory of a common ancestor for all life on Earth; because these core components are necessary for basic cellular functions and are found across all living organisms, indicating a shared evolutionary origin. Key characteristics shared by all cells: Plasma membrane: A selectively permeable outer boundary that separates the cell's internal environment from its surroundings, controlling what enters and exits the cell. Cytoplasm: A gel-like substance that fills the cell interior, providing a medium for cellular reactions and housing the cell's organelles. Ribosomes: Small, non-membrane bound structures responsible for protein synthesis, translating genetic information into functional proteins. DNA (Deoxyribonucleic Acid): The genetic material of all cells, carrying the instructions for cellular functions and traits, organized into chromosomes. Basic metabolic processes: Essential chemical reactions occurring within the cell, including energy production (ATP synthesis), nutrient breakdown, and biosynthesis of cellular components. Significance of shared traits in supporting a common ancestor: Universality: The presence of these core components in all known life forms, from single-celled bacteria to complex multicellular organisms, indicates a common evolutionary origin. Functional necessity: Each of these components plays a crucial role in basic cellular functions like replication, growth, and response to stimuli, making them essential for any living organism to survive. Molecular similarity: The building blocks of DNA, RNA, and proteins are remarkably consistent across all life, further supporting the idea of a shared ancestral genetic code. 10. Number of domains of life based on rRNA phylogeny: The current scientific consensus is that life is divided into three domains: Bacteria, Archaea, and Eukarya, with this classification primarily based on comparisons of small subunit ribosomal RNA (SSU rRNA) sequences, which reveal significant genetic differences between these groups, allowing scientists to construct a robust evolutionary tree of life. Explanation: SSU rRNA as a molecular clock: Ribosomal RNA is a crucial component of ribosomes, the cellular machinery responsible for protein synthesis, making it highly conserved across all living organisms. By comparing the nucleotide sequences of the SSU rRNA gene, scientists can infer evolutionary relationships between different species. Distinctive sequence patterns: Carl Woese, a pioneer in this field, discovered that the SSU rRNA sequences of organisms could be grouped into three distinct clusters, which formed the basis for the three domains of life: Bacteria, Archaea, and Eukarya. Evolutionary implications: The differences in SSU rRNA sequences between these domains indicate that they diverged very early in the history of life, with Archaea often considered to be more closely related to Eukarya than to Bacteria. Key points about the three domains: Bacteria: Typically single-celled prokaryotes with diverse metabolic capabilities, found in a wide range of environments. Archaea: Also prokaryotic, but often inhabit extreme environments like hot springs or deep- sea vents, with unique genetic and biochemical characteristics. Eukarya: Includes all organisms with complex cells containing a nucleus, encompassing plants, animals, fungi, and protists. 11. Differences between archaea and eukarya: Archaea and Eukarya are distinct domains of life, with the primary difference being that Eukarya possess membrane-bound organelles, a complex nucleus, and a more intricate cellular structure, while Archaea lack these features and are considered prokaryotic, sharing more similarities with bacteria in terms of cell organization, but with unique membrane lipid composition that sets them apart from both bacteria and eukaryotes; additionally, Eukarya often have histones associated with their DNA, which is not always the case in Archaea, and both groups exhibit differences in their translation machinery. Key Differences: Membrane-Bound Organelles: Eukarya have a well-developed nucleus and numerous membrane-bound organelles like mitochondria, endoplasmic reticulum, and Golgi apparatus, whereas Archaea lack any membrane-bound organelles. Cell Wall Composition: While some Archaea may have cell walls, they do not contain peptidoglycan, a key component of bacterial cell walls. Eukarya can have cell walls depending on the organism (e.g., plants have cellulose-based cell walls), but these are structurally different from those found in bacteria and Archaea. Membrane Lipids: A defining characteristic of Archaea is their unique cell membrane lipids composed of isoprenoid hydrocarbons linked to glycerol by ether linkages, which are not found in Eukarya (which primarily use ester linkages). DNA Organization: Eukaryotic DNA is typically linear and organized within a nucleus, often wrapped around histone proteins, while Archaea usually have a single circular chromosome located in a nucleoid region without histones in most cases. Translation Machinery: Although some similarities exist, the translation machinery (ribosomes and associated proteins) in Archaea is distinct from that of Eukarya, with certain subunits showing closer evolutionary relationships to Eukaryotic counterparts. Other Distinguishing Features: Extremophiles: Many Archaea are extremophiles, thriving in extreme environments like hot springs, deep-sea vents, or highly saline lakes, due to their unique membrane adaptations. Genetic Diversity: While some Archaea may exhibit a degree of genetic similarity to Eukarya in certain genes, overall, their genetic makeup is more distinct. Summary: Eukarya: Complex cells with membrane-bound organelles, linear DNA with histones, diverse cell wall compositions depending on the organism, ester-linked membrane lipids. Archaea: Prokaryotic cells lacking membrane-bound organelles, often with unique ether- linked lipids in their membranes, no peptidoglycan in cell walls, and sometimes sharing genetic similarities with Eukarya. 12. Criteria for defining a prokaryotic species: Outline the criteria used to define a prokaryotic species, including: SSU rRNA sequence similarity (generally ≥95%), average nucleotide identity (ANI) of orthologous genes (generally ≥95%), shared ecotype, and phenotypic characteristics between organisms with 95% or greater identity. A prokaryotic species is typically defined by a high degree of similarity in its small subunit ribosomal RNA (SSU rRNA) sequence, usually exceeding 95% identity, and a high Average Nucleotide Identity (ANI) of orthologous genes, with a value around 95% often considered the threshold for species delineation; essentially meaning that strains with such high sequence similarity are considered to belong to the same species. Key points about prokaryotic species definition: SSU rRNA sequence similarity: This is the most widely used criterion, where a high percentage of sequence identity in the 16S rRNA gene indicates close evolutionary relationship and potential species membership. ANI of orthologous genes: This method compares the nucleotide identity of shared genes between two genomes, providing a more comprehensive picture of genomic similarity beyond just the rRNA sequence. Threshold values: A commonly accepted threshold for species delineation is around 95% sequence similarity for both SSU rRNA and ANI. Important considerations: DNA-DNA hybridization: Historically, a 70% DNA-DNA hybridization value was used as a standard for species definition, but this method is less commonly used today due to technical challenges. Phenotypic characteristics: While not the primary determinant, phenotypic features like morphology and metabolic capabilities are still considered when defining a prokaryotic species Traditional species concepts based on interbreeding are not applicable to prokaryotes because prokaryotes primarily reproduce asexually through binary fission, meaning they do not exchange genetic material through sexual reproduction, which is the foundation of the "biological species concept" that defines species by their ability to interbreed with each other;therefore, using interbreeding as a criterion to classify prokaryotic species is not relevant. Key points about why the traditional species concept doesn't work for prokaryotes: Asexual reproduction: Prokaryotes primarily reproduce via binary fission, where a single cell divides into two identical copies, eliminating the opportunity for genetic exchange through sexual reproduction. Horizontal gene transfer: Bacteria can transfer genetic material between individuals through mechanisms like plasmids and conjugation, which further complicates the concept of distinct species boundaries. Genetic diversity within populations: Even within a single "species" of bacteria, there can be significant genetic variation due to high mutation rates and horizontal gene transfer, making it difficult to define clear species boundaries based on genetic similarity alone. 13. Definition of taxonomy: Taxonomy is the description of distinct life forms microbe isolated in pure culture and their organization into different categories with shared traits; it includes: Classification = the recognition of different classes of life Nomenclature = the naming of different classes Identification = the recognition of the class of a given Classification generates a hierarchy of taxa. Long-studied organisms tend to have many ranks, whereas recent isolates have few. Domain Kingdom Phylum Class Order Family Genus Species The hierarchical system of classification, also called taxonomy, organizes living organisms into groups based on their shared characteristics, starting with the broadest category "Domain" and becoming progressively more specific through Kingdom, Phylum, Class, Order, Family, Genus, and finally Species, allowing scientists to identify and categorize organisms based on their evolutionary relationships; essentially, the higher the level in the hierarchy, the more diverse the group of organisms within it, while the lower levels represent more closely related organisms. Key points about the classification hierarchy: Domain: The most inclusive level, currently divided into Bacteria, Archaea, and Eukarya, which represent major evolutionary lineages. Kingdom: A large grouping of organisms with significant shared characteristics, like Animalia, Plantae, Fungi, Protista, and Monera. Phylum (or Division in plants): A further division within a Kingdom, grouping organisms with similar body plans or developmental patterns. Class: A smaller grouping within a Phylum, with organisms sharing more specific characteristics. Order: A division within a Class, containing organisms with even more similar traits. Family: A group of closely related genera with shared characteristics. Genus: A group of closely related species sharing a common ancestry. Species: The most specific level, representing a group of organisms that can interbreed and produce fertile offspring. How it is used: Scientific Naming: Each organism is given a unique scientific name consisting of its Genus and Species, which allows for consistent identification across different languages and regions. Understanding Relationships: By classifying organisms together, scientists can infer evolutionary relationships between different species. Studying Biodiversity: By grouping organisms, scientists can better analyze and understand the diversity of life on Earth. 14. Example of endosymbiosis: Endosymbiosis refers to a symbiotic relationship where one organism lives inside another, mutually benefiting from the partnership; a prime example is the theory that mitochondria and chloroplasts within eukaryotic cells originated from engulfed prokaryotic cells, becoming organelles within the host cell over evolutionary time. Evidence supporting the endosymbiotic theory: Double membranes: Both mitochondria and chloroplasts have a double membrane structure, with the inner membrane resembling a prokaryotic cell membrane, suggesting they were once independent organisms engulfed by the host cell. Own DNA: These organelles possess their own circular DNA, similar to prokaryotic DNA, which replicates independently from the host cell's nuclear DNA. Ribosomes: The ribosomes found within mitochondria and chloroplasts are more similar to prokaryotic ribosomes than to those in the eukaryotic cytoplasm. Size and shape: Mitochondria and chloroplasts are roughly the same size and shape as many free- living prokaryotic bacteria. Binary fission: Both organelles divide by binary fission, a method of cell division typical of prokaryotes. Specific example: Mitochondria: According to the endosymbiotic theory, mitochondria likely originated from aerobic bacteria that were engulfed by a primitive eukaryotic cell. The host cell gained the ability to efficiently produce ATP through aerobic respiration, while the bacteria gained a protected environment to live within. Chloroplasts: Similarly, chloroplasts are thought to have originated from cyanobacteria, photosynthetic prokaryotes, which were engulfed by a eukaryotic cell, allowing the host to gain the ability to perform photosynthesis. Key points: The endosymbiotic theory explains the presence of complex organelles like mitochondria and chloroplasts within eukaryotic cells. The shared characteristics between these organelles and prokaryotes, such as double membranes, their own DNA, and similar size, strongly support the theory of their prokaryotic origin. 15. Ancestral organism of chloroplasts: According to the endosymbiotic theory, the most likely ancestral organism that gave rise to chloroplasts is a cyanobacterium; this photosynthetic bacteria was engulfed by a eukaryotic host cell, eventually becoming integrated as chloroplasts within the host, allowing the host to perform photosynthesis through this symbiotic relationship. Explanation of the endosymbiosis process: Engulfment: An early eukaryotic cell, likely lacking the ability to photosynthesize, engulfed a free-living cyanobacterium through a process similar to phagocytosis. Survival and Integration: Instead of being digested, the engulfed cyanobacterium remained viable within the host cell, gradually losing some of its independent functions over time. Gene Transfer: Genetic material from the cyanobacterium was transferred to the host cell's nucleus, allowing for coordinated function between the two organisms. Evolution of Chloroplasts: The engulfed cyanobacterium evolved into a specialized organelle, the chloroplast, with a double membrane structure - one derived from the cyanobacterium's cell membrane and another from the host cell's membrane. Evidence supporting the endosymbiotic origin of chloroplasts: Genetic Similarity: Chloroplasts have their own DNA, which is structurally similar to bacterial DNA, particularly that of cyanobacteria. Division Mechanism: Chloroplasts divide by binary fission, a process characteristic of prokaryotes like bacteria. Photosynthetic Pigments: Chloroplasts contain the same photosynthetic pigments as cyanobacteria, allowing them to capture light energy for photosynthesis. 16. Flagellation: Define and illustrate flagellation, describing the arrangement of flagella Flagellar Arrangements Monotrichous –single flagellum at one end Lophotrichous –small bunches emerging from the same site Amphitrichous – flagella at both ends of cell Peritrichous – flagella dispersed over surface of cell Flagella Rotate 360o Function in motility of cell through environment Involved in chemotaxis, which involves a response to chemical signals from attractants and repellants Chemotaxis is the movement of a bacterium in response to chemical gradients. Attractants cause CCW rotation. - Flagella bundle together Push cell forward - “Run” Repellents cause CW rotation. - Flagellar bundle falls apart. - “Tumble” - Bacterium briefly stops, then changes direction. 17. Bacteria shapes: Describe and illustrate the spherical shape of a coccus bacterium. Mention variations like diplococci, streptococci, staphylococci, and tetrads. A coccus bacterium is a spherical-shaped bacteria, appearing like tiny balls under a microscope; however, depending on how they divide and remain attached to each other after cell division, they can form different arrangements like diplococci (pairs), streptococci (chains), staphylococci (grape-like clusters), and tetrads (groups of four in a square arrangement). Variations: Single Coccus: A simple, individual spherical cell. Diplococcus: Two cocci attached together, appearing as a pair. Streptococcus: A chain of cocci, where each cell divides and remains attached to the previous one, forming a line. Staphylococcus: Irregular clusters of cocci, resembling a bunch of grapes, where cells divide in random planes and stick together. Tetrad: A group of four cocci arranged in a square, resulting from division in two perpendicular planes. 18. Prokaryotic nature of bacteria and archaea: Both bacteria and archaea are classified as prokaryotes, meaning they lack a nucleus and other membrane-bound organelles within their cells; however, a key difference lies in the composition of their cell membranes, with bacteria having ester-linked fatty acids while archaea possess ether-linked isoprenoid lipids, making the archaeal membranes more resistant to extreme environments. Key points about bacteria and archaea as prokaryotes: No nucleus: Both bacteria and archaea lack a defined nucleus to house their genetic material, which is instead located in a region called the nucleoid. No membrane-bound organelles: Neither bacteria nor archaea have internal organelles like mitochondria or endoplasmic reticulum, which are enclosed by membranes in eukaryotic cells. Differences in lipid linkages: Bacteria: The lipids in bacterial cell membranes are primarily composed of fatty acids attached to glycerol by ester linkages. Archaea: In contrast, archaeal lipids are made up of isoprenoid chains (branched hydrocarbons) linked to glycerol through ether linkages, which provide greater stability in extreme conditions. Cell membrane structure comparison: Bacteria: The bacterial cell membrane is a typical phospholipid bilayer with ester-linked fatty acids, creating a relatively standard membrane structure. Archaea: Archaeal membranes can vary depending on the species, but they often have unique lipid compositions like isoprenoid lipids with ether linkages, and some archaea may even have lipid monolayers instead of bilayers. 19. Relationship between magnification and image quality: The most important property for lens magnification is its focal length; a longer focal length results in greater magnification, allowing for a larger image of the specimen when viewed through the lens. Magnification of a light microscope: A light microscope achieves magnification by combining the magnification power of the objective lens and the eyepiece lens; the total magnification is calculated by multiplying the power of each lens together. How staining helps visualize bacteria: Staining enhances the visibility of bacteria under a microscope by adding color to the cells, which increases the contrast between the bacteria and the surrounding background, making them easier to see. Explanation of contrast enhancement through staining: When a dye is applied to a bacterial cell, it binds to specific components of the cell, creating a color difference between the cell and the unstained background, thereby increasing contrast and allowing for better observation under a microscope. Different types of stains: Simple stain: Adds dark color specifically to cells but not to the external medium or surrounding tissue. Methylene blue is the most commonly used stain. Uses only one dye, which stains all cells in the sample the same color, providing basic information about cell morphology (shape and arrangement). Differential stain: Stains one kind of cell but not another. Utilizes multiple stains to differentiate between different types of bacteria based on their cell wall characteristics. The most common example is the Gram stain, which distinguishes between Gram- positive and Gram-negative bacteria. 20. Advantage of a wet mount: A key advantage of a wet mount is that it allows you to observe living organisms in their natural state, enabling the study of their motility (movement) and dynamic cellular processes, as the specimen is suspended in a liquid medium on a microscope slide, unlike a stained slide which kills the organism and fixes it in place. Key points about wet mounts: Observe live organisms: You can see cells moving and interacting in real-time, which is crucial for studying behaviors like swimming, crawling, or phagocytosis. Simple preparation: Wet mounts are quick and easy to prepare, requiring minimal equipment and no complex staining procedures. Preserves natural state: Since no chemical fixation is involved, the specimen appears in its natural condition without distortion. Disadvantages include little contrast between cell and background and the sample my dry out 21. Complex formed in Gram-positive cells during Gram staining: During Gram staining, Gram-positive cells form a "crystal violet-iodine complex" within their cell walls, which is retained due to the presence of a thick peptidoglycan layer that effectively traps this complex, causing the cells to appear purple under the microscope; this is in contrast to Gram-negative bacteria which have a thin peptidoglycan layer and easily lose the complex during the decolorization step, taking up the counterstain instead. Key points about the crystal violet-iodine complex and Gram-positive cells: Formation: When crystal violet (primary stain) is applied to bacteria, it binds to the cell wall.Subsequently, the addition of iodine acts as a mordant, forming a large, insoluble complex with the crystal violet inside the cell. Thick peptidoglycan layer: Gram-positive bacteria have a thick, multilayered peptidoglycan cell wall, which acts as a barrier that prevents the crystal violet-iodine complex from being washed away during the decolorization step with alcohol. Decolorization effect: When decolorizer is applied, it dehydrates the thick peptidoglycan layer of Gram- positive cells, causing the pores to shrink and tightly trap the crystal violet-iodine complex within the cell wall. In summary, the crystal violet-iodine complex forms within the cell wall of Gram- positive bacteria due to the interaction between the crystal violet stain and iodine, and the thick peptidoglycan layer of these cells prevents the complex from being washed away during decolorization, resulting in a purple stain appearance. 22. Principle of phase-contrast microscopy: Phase-contrast microscopy enhances the visibility of transparent specimens by manipulating light waves, creating contrast based on slight differences in the refractive index between different parts of the specimen and its surrounding medium; it does this by splitting the light beam into two paths, one passing directly through the specimen and the other passing through a phase plate, causing a phase shift in the light that is then recombined to produce a visible image with enhanced contrast between different structures within the specimen. How contrast is created: Refractive index differences: Different parts of a specimen, like cell organelles or boundaries between the cell and its surrounding medium, have slightly different refractive indices, causing variations in the phase shift of light passing through them. Amplified phase shift: The phase plate further amplifies these small phase differences, making them readily visible as variations in brightness in the final image. Dark against bright background: Areas of the specimen with a higher refractive index appear darker against a brighter background due to the destructive interference of the light waves. 23. Microscopy Definition of magnification: Define magnification as the apparent increase in the size of an object. Magnification means an increase in the apparent size of an image to resolve smaller separations between objects. Magnification refers to the apparent increase in the size of an object, meaning how much larger an object appears to be when viewed through a lens or optical instrument, compared to its actual size; essentially, it's the process of enlarging an object visually without changing its physical dimensions. 24. Testing Koch's third postulate: Koch's third postulate states that to confirm a microbe as the cause of a disease, you must take the suspected pathogen, grown in a pure culture (meaning only that specific microbe is present), and introduce it into a healthy host, observing if the host develops the same disease symptoms as seen in the original infected individual; therefore, in the context of the question, the correct action would be to inject the bacteria into a healthy frog to see if it develops the disease. Koch’s postulates are an ordered set of criteria for establishing a causative link between an infectious agent and a disease. 1. Suspect microbe always present in diseased host – absent in healthy hosts 2. Suspect microbe grown in pure culture outside host – no other microbes present in culture 3. Cultured microbe introduced into healthy host – individuals become sick with same disease as original hosts 4. Same microbial suspect re-isolated from sick host 25. Carl Woese's contribution to classification: Carl Woese revolutionized the classification of life by using comparisons of small subunit ribosomal RNA (SSU rRNA) sequences to identify three distinct domains of life: Bacteria, Archaea, and Eukarya, effectively replacing the older five-kingdom system, as his research revealed that organisms previously grouped as "prokaryotes" were actually significantly different enough to warrant separate domains, with Archaea being the newly identified group distinct from both Bacteria and Eukarya; this groundbreaking discovery was made possible by analyzing the conserved rRNA sequences which act as a molecular clock, allowing for accurate evolutionary relationships to be determined between organisms. Key points about Woese's work: SSU rRNA as a marker: Woese recognized that SSU rRNA genes are present in all living organisms, highly conserved (meaning they change slowly over time), and can be used to compare genetic relationships across diverse species. Discovery of Archaea: By analyzing rRNA sequences, Woese discovered that a group of organisms previously considered bacteria, now known as Archaea, were actually more closely related to Eukarya than to Bacteria, leading to the three-domain system. Phylogenetic tree: Using rRNA sequence data, Woese constructed a phylogenetic tree that visually represented the evolutionary relationships between different life forms, clearly showing the distinct lineages of Bacteria, Archaea, and Eukarya. Impact on taxonomy: Woese's findings fundamentally changed the way scientists classify life, replacing the older five-kingdom system with the more accurate three-domain system.